Life: Difference between revisions
imported>Anthony.Sebastian (→Systems view of 'living' and the phenomenon of emergence: Adding content) |
imported>Anthony.Sebastian |
||
Line 44: | Line 44: | ||
::::::::::::<font color="purple">'''''Every object that biology studies is a system of systems.''''' '''''--Francois Jacob, The Logic of Living Systems: a History of Heredity (1974) ISBN 0713903600'''''</font> | ::::::::::::<font color="purple">'''''Every object that biology studies is a system of systems.''''' '''''--Francois Jacob, The Logic of Living Systems: a History of Heredity (1974) ISBN 0713903600'''''</font> | ||
::::::::::::<font color="purple">'''''What is life?...'Where is the program of life?'...there is no such program and...there is no privileged level of causality in biological systems.''''' '''''--</font> [http://www.us.oup.com/us/catalog/general/subject/LifeSciences/~~/dmlldz11c2EmY2k9OTc4MDE5OTIyODM2Mg== Denis Nobel | ::::::::::::<font color="purple">'''''What is life?...'Where is the program of life?'...there is no such program and...there is no privileged level of causality in biological systems.''''' '''''--</font> [http://www.us.oup.com/us/catalog/general/subject/LifeSciences/~~/dmlldz11c2EmY2k9OTc4MDE5OTIyODM2Mg== Denis Nobel (2006) The Music of Life: Biology Beyond the Genome. Oxford University Press.] ISBN 978-0-19-929573-9 | ||
[[Image:Acrosome_reaction_diagram_svg.png|thumb|400px|left|Oocyte and spermatozoon merging to begin a new living system.]] | [[Image:Acrosome_reaction_diagram_svg.png|thumb|400px|left|Oocyte and spermatozoon merging to begin a new living system.]] | ||
The evolutionary biologist [[Ernst Mayr]] suggested that, to define 'life', we need to clarify what we mean by the process of 'living': | The evolutionary biologist [[Ernst Mayr]] suggested that, to define 'life', we need to clarify what we mean by the process of 'living': | ||
Line 65: | Line 68: | ||
Philosopher of science D.M. Walsh puts it this way: "The constituent parts and processes of a living thing are related to the organism as a whole by a kind of 'reciprocal causation'."<ref>Walsh DM (2006) Organisms as natural purposes: the contemporary evolutionary perspective. Stud Hist Philos Biol Biomed Sci 37: [http://dx.doi.org/doi:10.1016/j.shpsc.2006.09.009 771-91]</ref> In other words, the organization of the components determine the behavior of the system, but that organization arises from more than the set of its internal components. How the whole system behaves as it interacts with its environment determines how those components organize themselves, and so novel properties of the system 'emerge' that characterize neither the environment nor that set of internal components. For example, the behavior of a human kidney cell depends not only on its cellular physiology, but also on all the properties of the organ ([[kidney]]) which constitutes its environment. The kidney's overall structure and function influence the cell’s structure and behavior (e.g., by physical confinement and by cell-to-cell signaling), which in turn influence the organization of its intracellular components. The kidney in turn responds to ''its'' environment, namely the individual body that it lives in, and that body responds to ''its'' environment, which includes such factors as the availability of particular food items, fresh water, and ambient [[temperature]] and [[humidity]]. Systems biologists regard emergent properties as arising from from a combination of ''bottom-up'' and ''top-down'' effects — Walsh's 'reciprocal causation'. | Philosopher of science D.M. Walsh puts it this way: "The constituent parts and processes of a living thing are related to the organism as a whole by a kind of 'reciprocal causation'."<ref>Walsh DM (2006) Organisms as natural purposes: the contemporary evolutionary perspective. Stud Hist Philos Biol Biomed Sci 37: [http://dx.doi.org/doi:10.1016/j.shpsc.2006.09.009 771-91]</ref> In other words, the organization of the components determine the behavior of the system, but that organization arises from more than the set of its internal components. How the whole system behaves as it interacts with its environment determines how those components organize themselves, and so novel properties of the system 'emerge' that characterize neither the environment nor that set of internal components. For example, the behavior of a human kidney cell depends not only on its cellular physiology, but also on all the properties of the organ ([[kidney]]) which constitutes its environment. The kidney's overall structure and function influence the cell’s structure and behavior (e.g., by physical confinement and by cell-to-cell signaling), which in turn influence the organization of its intracellular components. The kidney in turn responds to ''its'' environment, namely the individual body that it lives in, and that body responds to ''its'' environment, which includes such factors as the availability of particular food items, fresh water, and ambient [[temperature]] and [[humidity]]. Systems biologists regard emergent properties as arising from from a combination of ''bottom-up'' and ''top-down'' effects — Walsh's 'reciprocal causation'. | ||
Other basic concepts that systems biologists consider crucial in explaining living systems as-a-whole include 'robustness', 'modularity', and 'networks' — all discussed in sections below. Quantitative modeling and simulation guided by experimental biological data provide the mainstay methodologies of systems biologists. (See [[Systems biology]] and Denis Noble's 2006 on systems biology written for the general reader.<ref name=nobel06bk>Nobel D. (2006) [http://www.us.oup.com/us/catalog/general/subject/LifeSciences/~~/dmlldz11c2EmY2k9OTc4MDE5OTIyODM2Mg== The Music of Life: Biology Beyond the Genome.] Oxford University Press, New York. ISBN 978-0-19-929573-9 [http://www.dpag.ox.ac.uk/academic_staff/denis_noble Brief Biography] [http://books.google.com/books?id=0TRl4gsKYXMC&dq=%22denis+noble%22+%22music+of+life%22&printsec=frontcover&source=web&ots=0P20oX8q-Y&sig=rJ-8xkC4SSPAf346NnoAAlACSX4 Multiple Chapter Excerpts Online]</ref>) | Other basic concepts that systems biologists consider crucial in explaining living systems as-a-whole include 'robustness', 'modularity', and 'networks' — all discussed in sections below. Quantitative modeling and simulation guided by experimental biological data provide the mainstay methodologies of systems biologists. (See [[Systems biology]] and Denis Noble's 2006 book on systems biology, ''The Music of Life'', written for the general reader.<ref name=nobel06bk>Nobel D. (2006) [http://www.us.oup.com/us/catalog/general/subject/LifeSciences/~~/dmlldz11c2EmY2k9OTc4MDE5OTIyODM2Mg== The Music of Life: Biology Beyond the Genome.] Oxford University Press, New York. ISBN 978-0-19-929573-9 [http://www.dpag.ox.ac.uk/academic_staff/denis_noble Brief Biography] [http://books.google.com/books?id=0TRl4gsKYXMC&dq=%22denis+noble%22+%22music+of+life%22&printsec=frontcover&source=web&ots=0P20oX8q-Y&sig=rJ-8xkC4SSPAf346NnoAAlACSX4 Multiple Chapter Excerpts Online]</ref>) | ||
====Note on use of words 'organize' and 'organization'==== | ====Note on use of words 'organize' and 'organization'==== |
Revision as of 14:52, 24 August 2007
Biologists use the word life in several of its many senses: to refer to (1) the ‘biography’ of a living thing (e.g., the life of a mountain gorilla), sometimes even after a living thing has died (e.g., the life of Albert Einstein); (2) living things in the aggregate (e.g., plant life); (3) the relationships among living things (e.g., the life of the forest); (4) biology-related sciences (e.g., she became a life scientist, specializing in plant physiology); (5) intellectual or imaginative activity (e.g., the life of the mind); (6) all of the living things past and present (e.g., evolution of the diversity of life); and, (7) the processes that characterize living things that distinguish them from non-living matter (e.g., life as a unique material system). The latter sense of ‘life’ biologists use when asking the perennial questions, “what is life?” and “what is the origin of life?”
Potentially we might find elsewhere in the universe the same kinds of processes that uniquely characterize living things on Earth, generating endless forms most similar, or most different, from life on Earth. Or, further foregoing anthropomorphism, we might find different kinds of processes generating entities we still would recognize as living, as ‘alive’. Here can make observations and draw a few conclusions to address the “what is life?” question only for life on Earth.
Can we conceive that non-living things could somehow acquire naturally those processes that uniquely characterize living things? If they did, as biologists generally contend, can we ‘discover’ how they did it? We leave that question for an origin of life article. Here we focus on ‘discovering’ the processes that uniquely characterize living things (on Earth), which origin-of-life researchers would need to know in order to have a target for the mechanisms that led to the transition from the non-living to the living. This article focuses not on 'life', the noun, but on 'living', the verb; on what activities living entities perform, and on the essential basic processes that enable their performance — the principles of life. [1] It takes, as its theme, “Life is what is common to all living things on Earth” (Christian De Duve).[2]
(see also Biology and Systems biology).
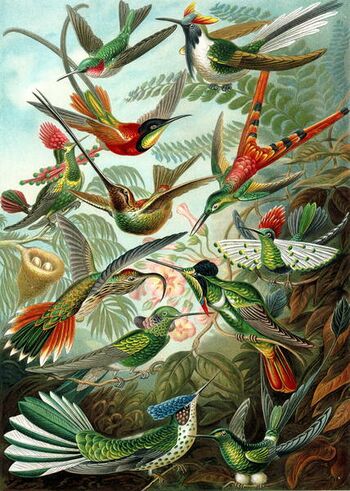
Principles of life
Molecules
- See related topics: Chemistry, Biochemistry, and Organic Chemistry
- From Molecule to Metaphor Jerome A. Feldman''
All known living things on Earth share a common set of types of organic molecules, including proteins, lipids, nucleic acids, and a host of other molecular genera. Although organic molecules contain many elements, they always have a predominant structure of carbon atoms, typically linked to themselves. In living things, organic species exist in non-homogeneous pools of colloidal aqueous solutions bounded by lipid and protein membranes. Each pool can have a different composition with distinct properties: transmembrane electrical potential; density; viscosity; osmotic pressure; and architecture. Those differences provide the basis for the generation of electric fields, fluid shifts, and transport of molecules into and out of the pools. The 'stuff' of life, then, is carbon-to-carbon chains, studded with other atoms, arranged in aqueous lagoons containing a variety of organic and inorganic molecules, interacting in accord with physico-chemical principles. (But see:[4])
Cells
- See Related Topics: Cell Biology, Microbiology
- Omnis cellula e cellula (Every cell out of a cell) —Rudolf Carl Virchow (1821–1902)
As well as sharing a common carbon- and water-based chemistry, every entity that biologists acknowledge as living — bacteria, trees, fish, chimpanzees — shares a common building block, the cell. Cells are universally enclosed in a membrane (a phospholipid bilayer known as the cytoplasmic membrane), that separates the inside of the cell from the external environment. Interestingly, the chemistry of the cell membrane is not universal. In most types of cells the molecules of the membrane are based on esters of glycerol combined with straight chain fatty acids, but in the Archaea the chemistry of membranes is based on glycerol ether linkages and isoprene fatty components.
Many organisms live as isolated single cells, others form cooperative colonies of cells, and still other cells are members of complex multicellular systems that include diverse cell types, each specialized for different functions.[5] Nature has produced an enormous variety of cell types that span three vast ‘domains’ of living systems: Archaea, Bacteria, and Eukarya,[6] yet cells in all three domains have many features in common. All cells have a surrounding membrane; a physical boundary that separates them from their environment (but see[7]) The surface of that membrane always has special properties that allow protection, excretion, ingestion or communication. Often, these functions are provided by changes in the shape or actual chemical species present on the surface — so pores, receptor molecules and protective walls are often features of the cell surface. This is true in both unicellular and multicellular entities.[8]
Current evidence indicates that only pre-existing cells can ‘manufacture’ cells. So how did the first cell(s) arise?
Examining what all extant cells have in common may provide insight to the Origin of life. All extract chemical energy from simple oxidation reactions, and convert it into other, chemical forms of energy. The molecule ATP universally serves as the cell's main energy 'currency'. All cells inherit digitally stored information in the form of molecules of DNA, and with minor exceptions the DNA of all cells use the same universal genetic code to guide production of a myriad of distinct protein structures. Cells use those proteins to carry out diverse activities, including energy processing and conversion of carbon, nitrogen and phosphorous-containing materials into cellular structures. In the human genome, perhaps as few as 22,000 different protein-coding genes[9] lead to the production of perhaps more than a million distinct protein structures that make up the variety and quantity of protein molecules needed for the structures and functions of a cell. Numerous molecular mechanisms account for that quantitative gene-to-protein amplification.[10]
Nature has produced a huge diversity of single-celled organisms and large, complex animals or plants. The latter can contain vast numbers of cells, each part of a specialized subpopulation (cell types) — in a mammal, the cells that make up bone differ from those that make up muscle, and differ again from those that make up skin, for example. Humans contain approximately 200 different cell types as classified by microscopic anatomy.[11] In multicellular organisms, cells combine to make organs, the functional and structural components of the single larger organism.
So what makes a single celled organism 'alive', and does the answer apply also when we call a large complex multicellular animal or plant 'alive'? What exactly do we mean by 'living'?
Systems view of 'living' and the phenomenon of emergence
(See main article, Systems biology)
- Every object that biology studies is a system of systems. --Francois Jacob, The Logic of Living Systems: a History of Heredity (1974) ISBN 0713903600
- What is life?...'Where is the program of life?'...there is no such program and...there is no privileged level of causality in biological systems. -- Denis Nobel (2006) The Music of Life: Biology Beyond the Genome. Oxford University Press. ISBN 978-0-19-929573-9
The evolutionary biologist Ernst Mayr suggested that, to define 'life', we need to clarify what we mean by the process of 'living':
- "The problem here is that 'life' suggests some 'thing' — a substance or force — and for centuries philosophers and biologists have tried to identify this 'vital force', to no avail. In reality, the noun 'life' is merely a reification of the process of living. It does not exist as an independent entity. One can deal with the process of living scientifically, something one cannot do with the abstraction 'life'. One can describe, and even try to define, what living is and what a living organism is, and one can try to make a demarcation between living and nonliving. Indeed, one can even try to explain how living as a process can be the product of molecules that themselves are not living."
The systems perspective of 'living' recalls Aristotle's four components of causality,[12] [13] in that a living thing comprises:
- A list of organic and inorganic parts (molecules and ions; cells, organelles, organs and organisms) — Aristotle’s 'material' cause;
- How the parts relate to each other to form structures (e.g., networks), how they interact with each other (e.g., network dynamics), and how the structures interact with each other in a coordinated dynamic and hierarchical manner — Aristotle’s 'formal' (form-like) cause;
- How the parts and structures became dynamically coordinated (e.g., gene expression; self-organization; competition) — Aristotle’s 'efficient' (effect-producing) cause; and
- How the living system as-a-whole functions and behaves, and the properties that characterize it (e.g., reproduction; locomotion; cognition) — Aristotle’s 'final' cause
The analysis of all of those components together forms part of the new discipline of Systems Biology. Systems biologists study, among other things, the phenomenon of 'emergence', whereby properties, functions, and behaviors of living systems arise though not exhibited by any individual component of the system, and not predictable from complete understanding the components' behaviors alone considered in isolation from the system that embeds them. Every cellular system exhibits emergent behaviors. Emergent behaviors of living systems include such things as locomotion, sexual display, flocking, and conscious experiencing.
Why can we not explain all of the properties of a system knowing the properties of its components? After all, the reductionist paradigm that dominated the Scientific method in the 20th century operated on the exactly opposite assumption. For one answer, the intrinsic, or individuated, properties of a system’s components of themselves do not determine those of the whole system; rather, their 'organizational dynamics' does, and those dynamics include not only the interrelations among the components themselves, but also interactions among the many different organizational units (subsystems) of the system. [14] For a second answer, the living system always operates in some context (its external environment, or surroundings), and those surroundings, in turn, always affect the properties of the system-as-a-whole. For example, nutrient gradients in a bacterium's environment influence the organization of the components of its locomotor apparatus and thereby the direction its locomotion. That exemplifies a way the impact of environmental context affects the organization of the components within the system — what systems biologists call 'downward causation'. [15] For another example, environmental signals can activate or suppress a metabolic pathway, reorganizing cellular activity[16] One cannot simply take a living system apart and predict how it will behave.
Philosopher of science D.M. Walsh puts it this way: "The constituent parts and processes of a living thing are related to the organism as a whole by a kind of 'reciprocal causation'."[17] In other words, the organization of the components determine the behavior of the system, but that organization arises from more than the set of its internal components. How the whole system behaves as it interacts with its environment determines how those components organize themselves, and so novel properties of the system 'emerge' that characterize neither the environment nor that set of internal components. For example, the behavior of a human kidney cell depends not only on its cellular physiology, but also on all the properties of the organ (kidney) which constitutes its environment. The kidney's overall structure and function influence the cell’s structure and behavior (e.g., by physical confinement and by cell-to-cell signaling), which in turn influence the organization of its intracellular components. The kidney in turn responds to its environment, namely the individual body that it lives in, and that body responds to its environment, which includes such factors as the availability of particular food items, fresh water, and ambient temperature and humidity. Systems biologists regard emergent properties as arising from from a combination of bottom-up and top-down effects — Walsh's 'reciprocal causation'.
Other basic concepts that systems biologists consider crucial in explaining living systems as-a-whole include 'robustness', 'modularity', and 'networks' — all discussed in sections below. Quantitative modeling and simulation guided by experimental biological data provide the mainstay methodologies of systems biologists. (See Systems biology and Denis Noble's 2006 book on systems biology, The Music of Life, written for the general reader.[18])
Note on use of words 'organize' and 'organization'
The verb 'organize' and noun 'organization' will appear frequently in this article, and therefore merit special note. When one organizes a collection of entities, one puts them in some kind of order, typically for functional purposes. Functionality serves as the criterion for organization, or for an organized state of the entities. Humans incline to organize things. They put their stamp collections in one or other kind of order to achieve the functionality of finding particular items, of determining what they have missing, of displaying their collection in a pleasing manner. They structure themselves in relation to one another in groups to achieve goals: sport teams, humanitarian societies, labor unions, congresses. To organize then implies to structure parts in relation to one another for functionality.
When it comes to living systems, nature too inclines to organization. Often she structures the parts in 'dynamic' relation, such that the parts 'interact' temporally. The parts work together in a 'coordinated' and 'hierarchical' way that achieves a functionality that contributes to the naturally selected goals of a living system to survive in the living state and to manufacture new living systems like itself. 'Dynamic', 'coordinated', 'hierarchical', and 'goal-directed functionality' characterize 'organization' in biological systems. The words 'organize' and 'organization' should invoke those properties. Thus 'organization' connotes more than 'order' when it comes to living systems — a special kind of order that achieves not only life-preserving functionality, but also unpredictable novelty, or emergent behavior.
Aristotle wanted to know how the parts became dynamically coordinated — how they became organized to achieve their final goal. As we shall see, nature achieves organization in living systems by enabling them to organize themselves. "There is grandeur in this view of life..." [19]
The thermodynamics of 'living'
- Organisms do not maintain their complexity, and become more complex, in a vacuum. Their high organization and low entropy is made up for by pollution, heat, and entropic export to their surroundings. --Eric D. Schneider and Dorion Sagan
Biologists often view living things from the perspective of thermodynamics — the science of interactions among energy (the capacity to do work), heat (thermal energy), work (movement through force), entropy (degree of disorder) and information (degree of order).[20] The sum total of these interactions define what a system can and cannot do when interconverting energy and work. For example, by the First Law of Thermodynamics, when a process converts one form of energy (e.g., light) to another (e.g., electricity; work), no net loss of energy and no net gain results.[21]
Scientists developed the laws of thermodynamics through experiment, debate, mathematical formulation and conceptual refinement; Albert Einstein believed that the laws of thermodynamics stood as an edifice of physical theory that would never topple. The 'Second Law of Thermodynamics has fundamental pertinence to the understanding of living systems:
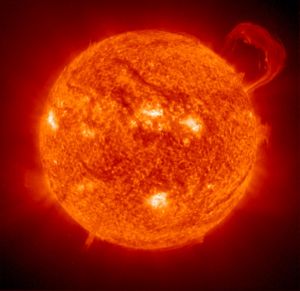
- Heat flows spontaneously — i.e., without help from an external agency or force — from a region of higher temperature to one of lower temperature, and never spontaneously in the reverse direction. That also holds for other forms of energy, including electromagnetic and chemical energy: concentrations of energy disperse, down-flow, to lower energy levels, flowing, so to speak, "into the cool".[22]
- When heat, as input to a system, causes it to perform work (e.g., as in a steam engine), it never converts entirely to work, an empirical fact. Some heat always dissipates as ‘exhaust’, unused and unusable by the system for further work. That also holds for other forms of energy doing work; some of the energy always turns into exhaust, typically heat unusable by the system doing the work. Energy conversion to work in a system can never proceed at 100% efficiency.
- Consequences arise from the fact that work can produce order in a system, but only by exporting heat to its surroundings. Experiments reveal the balance sheet of order: the degree of order of a system and its surroundings together never increases when energy causes the system to perform work; it always decreases — disorder increases. Scientists have learned how to quantify — put a number on — the degree of disorder of a system and surrounds, and they refer to that quantity as entropy.[23] Water vapor, with its molecules distributed nearly randomly, has a higher entropy than liquid water, with its molecules distributed less randomly, and a much higher entropy than ice, with its molecules distributed in a more ordered crystal array. Left to itself, ice tends to spontaneously melt and liquid water to evaporate. Order tends to disorder, with the Universe as a whole tending to exhaust itself into an ‘equilibrium’ state of randomness.
The above three bulleted expressions of the Second Law of Thermodynamics reflect the fact that energy and order spontaneously flow downhill — down a ‘gradient’, or descending ramp — toward eliminating the gradient of energy, as if nature abhors gradients of energy and order, as it abhors a vacuum.[22] Upon eliminating the gradient by flowing downhill, all energy and order has dissipated, all work production ceases, and an equilibrium state of maxikmal entropy ensues. Then how do living entities, those manifestly energetic organisms, manage to come into existence — to develop from an embryonic state to one of increasing order and decreasing entropy — and to perpetuate their order? How do they thwart the Second Law of Thermodynamics?
They don’t: they only seem to do so. Actually, they exploit the Universe’s downhill flowing gradients of energy and order. Like a steam engine, they 'import' energy and order, convert it, albeit incompletely, to the work of building internal order in the form of a dynamic organization of constituent elements, and so fabricate a system of decreasing their internal entropy. But all along they emit enough 'exhaust' to increase the disorder and entropy of their surroundings, so that the total entropy of the living system and its surroundings increase. Thereby they obey the Second Law.
Biological cells qualify as non-equilibrium thermodynamic systems. They consume the energy that flows through them, and use that energy to keep themselves from rampantly approaching the equilibrium state of randomness dictated by the Second Law. By managing to export unusable (degraded) energy in the form of heat and waste material, they actually export more disorder (entropy) than they produce within themselves, thereby increasing the total disorder (entropy) of the combined system and surroundings. Thus, they actually accelerate the dissipation of the energy gradient they find themselves in, as if nature's abhorence of energy gradients favored the development of living systems to maximize the rate of entropy production of the Universe as a whole. While maintaining the positive entropy balance of the Universe as a whole, living things can store energy and perform work both on themselves and their environment. When alive, a living system always performs its organizational work far from the 'equilibrium' state of activity that would ensue if no energy could be imported. Energy from outside supplies the driving force that keeps the system far from equilibrium. Non-equilibrium thermodynamic systems, including living things, can exhibit unexpectedly complex behaviors in virtue of their far from equilibrium state, and one very remarkable behavior that can result from this disequilibrum is self-organization.[24]
Some biophysicists propose that the production of order by matter in an energy gradient, as occurs in living things, tends to develop inevitably and to proceed inexorably. They give two reasons: (1) the production of order, by exporting more than counterbalancing disorder, increases total entropy production (i.e., dissipates the energy gradient and renders the dissipated energy unusable) beyond that which would otherwise occur, and (2) energy sources dissipate their gradient to produce disorder at the fastest rate possible — to reach equilibrium as fast as they can. In other words, the physical principles governing energy gradient dissipation and energy degradation not only allows the development of living systems, but, in effect, tends to select for them — or urges their emergence — in particular, when no constraints are present disallowing their development (e.g., excess heat, poverty of appropriate chemicals).[25] Thermodynamic principles thus may contribute not only to answering the question “what is life?” but also to “why is there life?”.[26] [27]
We can, then, view a living system as a state of organizational activity maintained by importing, storing and transforming energy and matter into the work and structures needed to sustain that state. They can only do so by producing waste and exporting it, and this lowers the ordered state of the environment. A living system maintains its organization at the expense of its external environment, leaving the environment more disordered than the gain in order of the living system — in keeping with the Second Law of Thermodynamics. Thus, from a thermodynamic perspective:
A living system has the ability to remain for a time in a quasi-steady-state as an organized system. The organization is made possible by the influx of energy and matter and by a more than compensatory efflux of waste (disorder), thereby allowing a far-from-equilibrium state to be maintained. |
Evolutionary aspects of 'living'
Last Paragraph of Charles Darwin’s Origin of Species (1859) "It is interesting to contemplate an entangled bank, clothed with many plants of many kinds, with birds singing on the bushes, with various insects flitting about, and with worms crawling through the damp earth, and to reflect that these elaborately constructed forms, so different from each other, and dependent on each other in so complex a manner, have all been produced by laws acting around us. These laws, taken in the largest sense, being Growth with Reproduction; Inheritance which is almost implied by reproduction; Variability from the indirect and direct action of the external conditions of life, and from use and disuse; a Ratio of Increase so high as to lead to a Struggle for Life, and as a consequence to Natural Selection, entailing Divergence of Character and the Extinction of less-improved forms. Thus, from the war of nature, from famine and death, the most exalted object which we are capable of conceiving, namely, the production of the higher animals, directly follows. There is grandeur in this view of life, with its several powers, having been originally breathed into a few forms or into one; and that, whilst this planet has gone cycling on according to the fixed law of gravity, from so simple a beginning endless forms most beautiful and most wonderful have been, and are being, evolved." |
Historically, fires and storms both have achieved status as living entities in human 'poetic' imagination. Although some non-living entities, such as tornadoes or the flames of candles, exist, like living things, as non-equilibrium open thermodynamic systems, they lack essential qualities of living things, and those deficiencies remove scientific credence of the 'poetic' view. Tornadoes and candle flames cannot 'reproduce' themselves, as cells and organisms can. Fire may spread and tornadoes may split, but the full system that comprises each phenomenon does not self-replicate. Living systems not only have open access to the environment in terms of energy and entropy exchange, but also have the capability of reproducing themselves. When a living system reproduces itself, its offspring inherit its properties, but with variations introduced by random events (including mutations). Some variations offer some of the offspring[28] less opportunity to reproduce than others, and other offspring better opportunity, sometimes better even than their parents. Accordingly, new groups with different properties arise that may supplant older groups because of their greater reproductive fitness. Biologists call this "evolution by natural selection", and many regard it as the most important way whereby living systems evolve over geological time.[29]
Therefore, biologists recognize the ability to produce offspring that inherit some of its features, but with some variation due to chance, as an essential characteristic of living systems. They refer to it as descent with modification, though other evolutionary forces contribute to such descent.[19] Evolution by natural selection will occur if heritable variations produce offspring that differ in their reproductive fitness. The variations occur due to chance variations in the inherited genetic recipe (genotype) for constructing the organismic traits (phenotype). In all living systems, DNA primarily provides the genetic recipe. All living things extant today descended with modification from an ancient ancestral community of microorganisms with a partially shareable gene pool. To glimpse beyond that horizon, we will need to take heed of the findings of intense current research on early cellular evolution (see Evolution of cells).
Viruses have few of the characteristics of living systems described above, but they do have a genotype and phenotype, making them subject to natural selection and evolution. Accordingly, descent with modification is not uniquely a characteristic of living systems. Beyond the scope of this article, we find descent with modification in memes and the artificial life of computer software, such as self-modifying computer viruses and programs created through genetic programming. Descent with modification has also been proposed to account for the evolution of the universe.[30]
Combining the thermodynamic and evolutionary perspectives, we might say that:
A living system has the ability to remain for a time in a quasi-steady-state as an organized system. The organization is made possible by the influx of energy and matter and by a more than compensatory efflux of waste (disorder), thereby allowing a far-from-equilibrium state to be maintained. A system is also capable of participating in the transgenerational evolution of the species to which it belongs in adapting to changing environments. |
The exobiologists' view
Exobiologists (also known as astrobiologists) consider issues relating to the possible existence of extraterrestrial living systems. Dirk Schulze-Makuch and Louis Irwin attempted to distill the essential characteristics of a living system in their book Life in the Universe.[31] They stress these characteristics, which resonate with the systems, thermodynamic and evolutionary perspectives discussed above:
- a microenvironment, with a boundary between it and its external environment,
- the ability of that microenvironment to transform energy and matter from the environment to maintain a highly ordered, 'organizational' state (a low entropy state),
- therefore, the ability of that microenvironment to remain in thermodynamic disequilibrium with its environment,
- the ability of that microenvironment to encode and transmit information.
Self-organization
In living systems, self-organization 'emerges' from the interactions among the systems' components. In cells, self-organization emerges in part from so-called supramolecular (non-covalent) interactions of proteins with proteins and other molecules.[32] [33] The proteins make their appearance through a genetic transcription-translation machinery, which itself represents a self-organized molecular machine that emerges in part from the non-covalent interactions of proteins and other molecules.[34] Molecules interact by forming and breaking strong or weak covalent bonds, and also through weaker, quasi-stable non-covalent electromagnetic interactions, like hydrogen bonding and van der Waals' forces. Those supramolecular interactions, and the flexibility of bond formation and breaking by weak covalent bonds, self-assemble aggregates of molecules (e.g., organelles, networks), giving them the physical properties that enable many biological processes.[33] [35] [36] [37]
Professor of Microbiology, Franklin M. Harold, offers the following definition of self-organization:
For the purposes of cell biology, let me define self-organization as the emergence of supramolecular order from the interactions among numerous molecules that obey only local rules, without reference to an external template or global plan...The definition explicitly excludes order imposed by an external template, whether physical (as in a photocopier) or genetic (as in the specification of an amino acid sequence by a sequence of nucleotides)...The structure of the self-assembled complex is wholly specified by the structures of its parts and is therefore implicit in the genes that specify those parts: natural selection crafted those genes to specify parts that assemble into a functional complex.[38]
Information resides in proteins and other molecules in virtue of their structure, and through them information flows through cells, just as energy does, and determines their organizational nature.[39]
One way to understand self-organization is to view the genetic information (the genome) as a 'computer program' that guides construction of the components of the cell that then arrange themselves according to their chemical properties. That arrangement, with the tinkering comprising local trial-and-error and evolution’s handiwork, can then carry out ('compute') integrative functions that are not explicitly encoded in the genome.[40] The molecular biologist Sidney Brenner[41] expressed the metaphor this way:
...biological systems can be viewed as special computing devices. This view emerges from considerations of how information is stored in and retrieved from the genes. Genes can only specify the properties of the proteins they code for, and any integrative properties of the system must be 'computed' by their interactions. This provides a framework for analysis by simulation and sets practical bounds on what can be achieved by reductionist models.[42]
The patterns of structure and behavior in self-organized systems need no behind-the-scene 'master controller', and no prepared recipes that specify the structure and dynamics of the system. Instead, those patterns emerge from the interactions among the naturally generated and selected components of a system, dictated by their physical properties, and dynamically modified by the emerging organization, which is itself modified by the environment. Thus the single-celled zygote self-organizes into a multicellular living system as the genetically encoded proteins interact, responding to changing influences from the changing environment generated by growing multicellularity — becoming a network of many cell-types working cooperatively. That biological systems self-organize has led one prominent biologist to say they are products of a "blind watchmaker".[43]
Self-organization tends to breed greater complexity of self-organization. One important aspect of self-organization in cells rests on the tendency for lipid molecules with polar (water-loving) and non-polar (water-shunning) ends to line up side-by-side to form bilayers in an aqueous solution, each unit of the bilayer with two lipid non-polar ends mutually attracted in the center and the polar ends surrounded by water. Membranes thereby form. Protein molecules can span the bilayer membrane, or selectively straddle only one or the other side of the membrane and its aqueous surrounding, according to their specific amino-acid sequence and side-groups. Other aspects of self-organization: Genes express not only proteins that organize themselves into a functional unit, but also proteins that organize themselves to regulate that unit, as in transcription regulatory circuits. Protein networks interact in a self-organizing way to produce networks of networks with complex levels of coordination, as in metabolic pathways. Cells communicate with other cells, either in free-living cellular communities or in multicellular organisms, and those communication activities self-organize by virtue of the properties of the cells, selected for fitness by evolutionary mechanisms, and subject to downward effects by the systems' organization and environmental influences on the systems.
Further elaborating the descriptions of living systems beyond the thermodynamic and evolutionary perspectives, we might say that:
A living system has the ability to remain for a time in a quasi-steady-state as a self-organized system. The organization is enabled by the influx of energy and matter and by a more than compensatory efflux of waste (disorder), thereby exploiting a far-from-equilibrium state. A system is also capable of participating in the transgenerational evolution of the species to which it belongs in adapting to changing environments. |
Autonomous agents
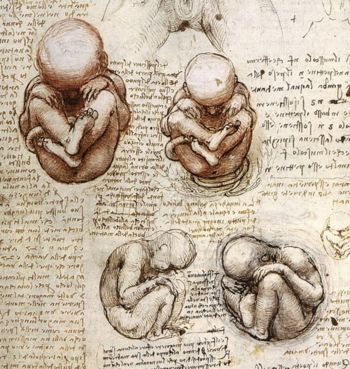
Stuart Kauffman uses the concept of 'autonomous agents' to explain living systems.[44][45] He gives the hypothetical example of an enzyme that catalyzes the binding of two smaller sub-component molecules into a copy of itself — self-replication by auto-catalysis. The energy to produce the enzyme comes from a neighboring molecule, by breaking an energy-rich bond, thus the neighbor molecule serves as a 'motor' to produce excess enzyme. The self-replication stops after using all duplicates of the motor, so to sustain self-replication, external energy — perhaps from light impinging on the system — must drive the repair of the broken chemical bond, re-establishing an ample supply of that energy-supplying molecule, thereby re-energizing the motor. A new cycle of auto-catalytic self-replication can then begin, given an ample influx of both external energy and 'food' (sub-components of the auto-catalytic enzyme). As an essential feature, interactions among the components of a system have effects (technically 'allosteric' effects) that help organize and coordinate its processes, allowing the self-replication to proceed.[44]
Kauffman conceives, then, of an autocatalytic molecule in a network of molecules that has cycles of self-replication driven by external energy and materials. The network has a self-replication process as a subsystem, and a motor, namely, the breakup of an energy-rich molecule, supplying energy that drives the self-replication, and its re-energizing repair by transduction of external energy. Such a network is a 'molecular autonomous agent' because, given external energy (e.g., photons) and ample materials (the molecules needed to assemble the autocatalytic enzyme), the network perpetuates its existence;. The network is autonomous because it is not controlled by outside forces even though it depends on outside energy and materials. The 'agent' is the system doing work autonomously; in this case, the work of auto-catalytic self-replication. (That's what 'agents' do; they do work.) In this example, the agent survives by ‘eating’ outside materials and energy. Work gets done because the system remains far-from-equilibrium: as energy flows through the system, the system does its work, and in so doing dissipates the energy gradient, but it temporarily constrains the rate of dissipation by storing energy in its internal organization. The agent continues to live (survive and self-replicate) only while that far-from-equilibrium state exists, and it can be starved to 'death' by stopping the matter and energy from flowing through the system. Kauffman argues that cells, and indeed all living systems, qualify as autonomous agents, constructed from molecular autonomous agents.[44]
Autonomous agents also interest scientists in the fields of artificial intelligence and artificial life. One careful description of autonomous agents from some members of that group adds further insight to this view of living systems:
"An autonomous agent is a system situated within and a part of an environment that senses that environment and acts on it, over time, in pursuit of its own agenda and so as to effect what it senses in the future. It has the properties of reactivity (timely response to environmental changes; autonomy (controls its own actions); goal-orientation (pursues its own agenda); continuous processing. Some autonomous agents may also have the properties of communicability (with other agents); adaptability (based on previous experience); unscripted flexibility."[46]
For Kauffman, the property of pursuing its own agenda includes contributing to its own survival and reproduction: "...an autonomous agent is something that can both reproduce itself and do at least one thermodynamic work cycle. It turns out that this is true of all free-living cells, excepting weird special cases. They all do work cycles, just like the bacterium spinning its flagellum as it swims up the glucose gradient. The cells in your body are busy doing work cycles all the time."[47] There is only one escape from work, and that is death.
If the descriptions of living systems from thermodynamic, evolutionary, self-organizational and autonomous agent perspectives are considered, we might say that:
A living system has the ability to remain for a time in a quasi-steady-state as a self-organized system. It works autonomously to offset responses to perturbations, and to reproduce itself, enabled by the influx of energy and matter and by a more than compensatory efflux of waste (disorder), thereby exploiting a far-from-equilibrium state. Finally, it is capable of participating in the transgenerational evolution of the species to which it belongs in adapting to changing environments. |
Networks
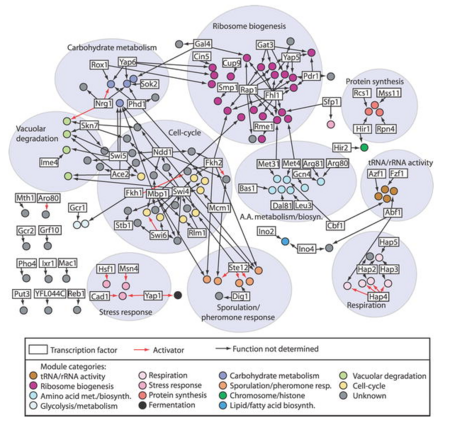
The science of networks[48] provides another useful perspective on living things. Networks ‘re-present’ a system as 'nodes’ and ‘interactions’ among the nodes (also referred to as ‘edges’ or ‘arrows’ or ‘links’). For example, in a spoken sentence, words and phrases make up the nodes, and the interconnections of syntax (subject-to-predicate, preposition-to-object of preposition, etc.) make up the links. Intracellular molecular networks represent specific functions in the cell; molecules make up the nodes, and their interactions with other nodes make up the edges or arrows. Some networks accept inputs of one kind and return outputs of a different kind.
One finds networks everywhere in biology, from intracellular signaling pathways, to intraspecies networks, to ecosystems. Humans deliberately construct social networks of individuals working (more or less) to a common purpose, such as the U.S. Congress; they also construct networks of electronic parts to produce, for example, mobile phones; and networks of sentences and paragraphs to express messages, including this very article. Researchers view the World Wide Web as a network, and study its characteristics and dynamics.[48][49]
According to Alon, "The cell can be viewed as an overlay of at least three types of networks, which describes protein-protein, protein-DNA, and protein-metabolite interactions."[50] Alon notes that cellular networks are like many human engineered networks in that they show 'modularity', 'robustness', and 'motifs':
- Modules comprise subnetworks with specific functions differing from those of other modules, and which typically but not invariably connect with other modules, often only at one input node and one output node. An individual module achieves its status as a distinct entity not only by its functional specificity but also by spatial specificity (e.g., ribosomes) or by chemical specificity (e.g., signal transduction networks). Modularity helps to facilitate real-time system adaptability to environmental change, as the organization of modules in the system contributes to the emergent properties of the system.[51] It also facilitates evolutionary adaption, as, to select an adaptation, evolution may need tinker with just a few modules rather than with the whole system. Evolution can sometimes 'exapt' existing modules for new functions that contribute to reproductive fitness. For example, Darwin surmised that the swim bladder of skeletally heavy fish evolved as an adaptation for control of buoyancy but was exapted as a respiratory organ in certain fish and in land vertebrates. [52][53]
- Robustness describes how a network is able to maintain its functionality despite environmental perturbations that affect the components. Robustness also reduces the range of network types that researchers must consider, because only certain types of networks are robust.[54]
- Network motifs offer economy of network design, as the same circuit can have many different uses in cellular regulation, as in the case of autoregulatory circuits and feedforward loops. Nature selects motifs in part for their ability to make networks robust, so systems use motifs that work well over and over again in many different networks.[55] In several well-studied biological networks, the abundance of network motifs — small subnetworks — correlates with the degree of robustness.[56] Networks, like those in cells and those in neural networks in the brain,[57] use motifs as basic building blocks, like multicellular organisms use cells as basic building blocks. Motifs offer biologists a level of simplicity of biological functionality for their efforts to model the dynamics of organized hierarchies of networks.[55]
The view of the cell as an overlay of mathematically-definable dynamic networks can reveal how a living system can exist as an improbable, intricate, self-orchestrated dance of molecules.[58] The 'overlay of networks' view also suggests how the concept of self-organized networks can extend to all higher levels of living systems.
Further elaborating the descriptions of living systems beyond the thermodynamic, evolutionary, self-organizational and autonomous agent perspectives we might say that:
A living system has the ability to remain for a time in a quasi-steady-state as a self-organized system of hierarchical robust modular networks. It works autonomously to offset responses to perturbations and to reproduce itself, enabled by the influx of energy and matter and by a more than compensatory efflux of waste (disorder), thereby exploiting a far-from-equilibrium state. Finally, it is capable of participating in the transgenerational evolution of the species to which it belongs in adapting to changing environments. |
Information processing
Bioscientists study biological systems for many different reasons, hence biology has many subdisciplines (see Biology and List of biology topics). But in every subdiscipline, bioscientists study biological systems for the proximate reason of gaining information about the system to satisfy their however-motivated curiosity and to apply that information to human agendas (e.g., to prevent disease, to develop treatments, to enhance health and longevity, to conserve the environment, etc.). Those realities attest that biological systems harbor information, at least as people usually understand the term. To appreciate how that perspective can contribute to understanding living systems, the following questions need answers:
- what do we mean by information?
- how does information apply to biological systems?
- how does information emerge in biological systems?
- how do the answers to those questions add to explaining living systems?
The word 'information' comes from the verb 'to inform', originally meaning to put form into something: the seal in-forms the wax, and the wax now contains in-formation. A random collection of particles or other entities has no form, nothing has given it form, and it contains no in-formation. The more randomness in the structure of the collection, the fewer improbable arrangements or interactions it has among its parts.
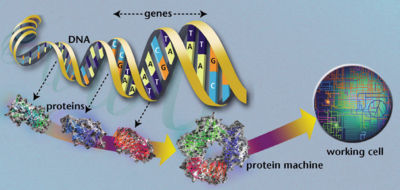
A drinking glass falls onto the sidewalk, it falls apart into a random collection of bits of glass. Notice it doesn’t regroup into the drinking glass — you could watch it for a lifetime. Our experience shows us that the drinking glass is more improbable than the glass smithereens. The more improbable the arrangements, the more in-formation a collection of parts has received and therefore contains. An observer will conclude that something has happened to form the parts into a more improbable state — an in-formation has occurred, and that the collection of parts contains that in-formation. By that reasoning, biological systems contain in-formation: something has happened to 'form' the parts into an improbable state.[59]
An ordered desktop soon becomes disordered. The ordered desktop has message value, or 'information', in that something must have happened to give it form. The more unlikely the arrangement of the parts, the more information it contains. Biological systems have information content in that they are unlikely (non-random) arrangements of parts, non-random collections of interactions of parts, and non-random collections of functional activities.
The above-discussed thermodynamic and autonomous agent perspectives viewed cells as interposed between a higher-to-lower degrees of usable (free) energy — embedded in downward sloping free energy gradient. The flow of energy through the cell fuels it, enabling it to perform the work that leads it to gain form, or order, or organization, and to gain functionalities, which raises its information content.[60]
Thus a living system emerges as an information processing system. It can receive information from energy[61] and energy-rich materials in its environment, which fuels and supplies the machinery that builds and sustains information-rich organization; it can generate new information inside itself, as in embryonic development; and it can transmit information within and outside itself, as in transcription regulation and exporting pheromones. From its parent(s), it inherits information (genetic) that provides a database to help it realize its developmental potential — including information critical for its self-reproduction, though it also inherits information in non-genetic forms that contribute to its development.Cite error: Closing </ref>
missing for <ref>
tag [62] [63]
Combined with other perspectives, viewing living systems as information processors, as inheritors, receivers, generators and transmitters of information, and as reproducers of inherited information, enables one to see living systems and their interactions with other living systems as a vast, complex, emergent, naturally-selected, self-sustaining, evolving communications network. Recently, on the timescale of evolving living systems, that evolving communications network emerged as the human brain, capable of communicating with itself and other humans using networks of symbols.[64] That led to the emergence of cultural evolution, a whole new domain of self-reproducing entities ('culturgens', 'memes') and a whole new domain of descent with modification. That in turn led to the emergence of other vast communications network: books, wikis, and other technologies of information generation and exchange.
We might now consider another closely related perspective, a ‘cognitive’ perspective.[65] Given that networks resist common perturbations (e.g., by their robustness, and by ‘homeostasis’), one might think of them as containing a representation of themselves and of their environment, and of how they might vary. As networks self-organize through interactions among proteins, any network-like 'representation’ of of the living system embedding it, and its environment, must derive from the information that determines those proteins. The genetic information comprises a molecular code, and the process that transforms that information into proteins describes an algorithm — the transcription-translation algorithm, including its regulatory circuits. Inasmuch as those algorithms evolved through natural experiment and selection, one can view evolution as selecting for cognitive functionality in the genome — the ability to ‘represent’ the cell’s state and environment and, more generally, to remember and anticipate.
Genetic information has the form of a digital code, one whose execution jump-starts cellular processes, including the processes that lead to self-organization of networks that regulate execution of the genetic digital code — the gene regulatory networks. A separate digital code also has a central role in the operation of those gene regulatory networks: the code adjacent to a gene determines which transcription regulating factors can bind there, and thereby controls gene activity. In other words, a digital code, separate from the code that specifies the proteins of the gene regulatory networks, gives specificity to the behavior of those networks and to their regulation of the execution of the genetic digital code.[66] Eventually, digital codes surrender to decipherment, offering the hope that we might someday read the message they contain and find ways to edit it.
Further elaborating beyond the thermodynamic, evolutionary, self-organizational, autonomous agent and network perspectives we might say that:
A living system has the informational content and information-processing faculty to remain for a time in a quasi-steady-state as a self-organized system of hierarchical robust modular networks. It works autonomously to offset responses to perturbations and to reproduce itself, enabled by the influx of energy and matter and by a more than compensatory efflux of waste (disorder), thereby exploiting a far-from-equilibrium state. Finally, it is capable of participating in the transgenerational evolution of the species to which it belongs in adapting to changing environments. |
Organic chemistry as informatics
Why the central place of carbon in the chemistry of all earth’s living things? Carbon has four electrons in its eight-electron-capacity outer shell, each of which readily seeks an additional electron to share with another atom, to form so-called covalent bonds with those other atoms. The physical chemistry of carbon thus enables it to bond with many other elements with unfilled outer shells. Those include hydrogen, which can share one electron with carbon to fill its outer shell, allowing carbon to covalently bond to four hydrogen atoms, as in methane (CH4) [=natural gas]; oxygen, which can share two electrons with carbon to fill its outer shell, allowing carbon to double-covalently bond with two oxygen atoms, as in carbon dioxide (CO2, or O=C=O; and nitrogen, which can share three electrons with carbon to fill its outer shell, allowing carbon to triple-covalently bond with one nitrogen atom, as in hydrocyanic acid (HCN). Most importantly, carbon can share electrons with itself, allowing the formation of carbon-to-carbon bonds, including carbon-to-carbon double bonds (C=C) and triple bonds. The avidity for carbon to bond to itself allows carbon atoms to easily join into long linear chains, with or without one or many carbon-to-carbon side chains, or even closed rings of carbon-to-carbon bonds, with or without side chains. Rings and chains and branches of linked carbons can combine into almost any form or shape one can imagine. The particular covalent bonding capacity of carbon thus enables it to combine with hydrogen, oxygen, nitrogen, and itself in multi-varied ways that generate small carbon-based molecules such as sugars, amino acids and nucleotides, which can join into huge macromolecules with remarkable stability under cellular conditions. The sequences of the varied subunits of such macromolecules give them the informational content required for constructing the dynamic organization of cells and for constructing copies of themselves.
The variety of carbon bonds vary in strength as well as in 3-D conformation; adding a dynamic quality to many organic molecules. The simplest set of bonds that carbon can form is that of a tetrahedron, or pyramid. The capacity of carbon for single, double and triple covalent bonding provide for a variety of geometries. Changing from one type of carbon-to-carbon bond to another type, as when a double bond is reduced to a single bond, will cause energy changes but without destroying the molecule. Such changes not only affect free energy, but also affect the actual shape of the molecule and the particular side groups attached to it. In this way, for at least some organic molecules, the 'pulse of life' is represented at an atomic level.
The properties of carbon mean that organic macromolecules are capable of containing tremendous 'banks' of information coded in their very structure. Not only can each of the constituent molecules be huge, but several categories of chemicals, like nucleotides or amino acids, that contain several different species, can be ordered so that the possible combinations are effectively limitless. All of these molecules are involved in the molecular-interaction networks of cells. Amongst these networks of interactions are those that enable cells to import and transform energy and energy-rich matter from the environment and that ultimately enable cells to grow, survive and reproduce.
Elsewhere in the universe, where conditions differ greatly from earth’s, other elements may actualize living systems. Silicon, carbon's close columnar relative on the periodic table, also forms bonds with itself, but they have little stability under conditions compatible with life as we know it. In places in the universe where physical conditions might favor silicon-based macromolecules, silicon-based life might exist. [67] Living systems, whether carbon-based or not, may not even require water to support the organization's chemistry. [68] (See also speculation about inorganic life in outer space plasma.[4])
Identifying the different scientific perspectives on life
The different perspectives biologists use in viewing living systems can be identified as follows:
- Living systems import energy, matter, and information from their environment, and export waste. This flow enables living systems to organize and maintain themselves, and thus to delay (for their lifetime) the dictate of the Second Law of Thermodynamics, that organized systems ultimately degrade to a state of randomness;
- The basic building blocks of all living systems are cells; the basic (genetic) information that generates cells comes as part of their starting materials. This information, in the form of nucleic acid macromolecules, encodes many different types of proteins that interact to assemble an organization that can import energy and export waste. Cells inherit this information from ‘parent’ cells, raising two as yet unanswered questions: how did cells arise in the first place? and how did they acquire stores of information?;[69] (see Origin of life and Evolution of cells)
- The molecular interactions are governed by the universal laws of physics and chemistry; those laws, together with the inherited information, enable a self-organizing system that can work autonomously for survival and reproduction, and allow properties to emerge that could not be anticipated from those of the system's components alone.
- The activities of a living system have no 'master controller'; they need only a type of organization that maintains the system far-from-equilibrium, which can yield improbable self-organized structures and activities.
- Living things cannot escape from changing external conditions, so they must exhibit robustness in their organization and must be adaptable to maintain their stability. Robustness and adaptability derive from the properties of a hierarchical network of subnetworks of molecular circuits;
- Living systems must produce enough reproductive variability to allow evolution through natural selection, which guides the continuation of a 3.5 billion year history of Earth’s living world. By evolution, living systems generate increasing varieties of living systems, occupy an extreme spectrum of environments, create their own environments,[70] and permit sufficient complexity to enable them to process information in a way that allows them to ‘experience’ themselves.
Synthesis of perspectives on what constitutes Life
The activity of living, for a cell-based system, depends on the ability to maintain a quasi-steady-state of organized functioning far from the state of randomness. The system achieves that in part because of its location in the path of a downhill gradient of flowing energy. It exploits that gradient through its abilities to import some of the energy flowing past it and to export unusable energy and material, in the process increasing its own order at the expense of a more than counterbalancing disorder of its surroundings.
Those principles seem to apply to all living systems: single cells, multicellular organs and organisms, and multi-organism demes and ecosystems. The fundamental challenges to staying alive do not differ greatly for an amoeba from those of a human. Neuroscientist Antonio Damasio[71] puts it this way:
All living organisms from the humble amoeba to the human are born with devices designed to solve automatically, no proper reasoning required, the basic problems of life. Those problems are: finding sources of energy; incorporating and transforming energy; maintaining a chemical balance of the interior compatible with the life process; maintaining the organism's structure by repairing its wear and tear; and fending off external agents of disease and physical injury.
The building block and working unit of all living systems is the cell. For cells to utilize available external energy or energy-rich matter to achieve and maintain a state of complex organization (order), they must have, from the outset, a basic informational content. That information enables the cell to produce components that can, by natural molecular interactions, respond to the imported energy and material to self-organize. That organization comprises modular networks of molecular interactions, and a hierarchy of interacting networks — self-organized and coordinated functional interactions. The properties of the networks and those of the hierarchy of networks enable the system to perpetuate itself, and to maintain its steady-state despite fluctuations in environmental factors. That principle, too, applies to all living systems. Any organism, plant or animal, comprises a network of organs working autonomously, maintaining its steady-state functioning far from equilibrium in response to environmental perturbations — physiologists refer to that as homeostasis.
The networks that regulate the flow of information through the cell resulted from natural experiments selected by natural selection and other evolutionary processes. That is, the codes that evolved by natural experiment and selection were those which produced molecules that could interact in ways that contribute to self-organization of those networks that enable a cell to sustain and reproduce itself. The collaboration of natural selection and physico-chemical laws perpetuates living systems not only in real-time but also in geological, or ‘evolutionary’, time. From common ancestors — however they may have arisen (see Evolution of cells) — informationally-guided, self-organizing, autonomous network dynamics enabled generation of the diversity of all living systems on the planet, over a period of more than three billion years. Living systems perpetuate living systems, exploiting free energy on its inexorable path to dissipation and degradation, and harvesting energy in developing systems organization by a more than counterbalancing dis-organizing of the larger system in which it is embedded.
Living machines: living systems as self-fabricating autonomous homeostatic cognitive machines
In this section we consider living systems, as distinct from non-living systems, from the perspective of the concept of ‘autopoiesis’ — autonomous self-fabrication — introduced in modern times by Humberto Maturana and Francesco Varela,[72] though first enunciated, as pointed out by J-H S. Hofmeyr,[73] by the philosopher Immanuel Kant.
Any entity we recognize as living we recognize also as a ‘system’, an assemblage of components, interrelated structurally, interacting in a coordinated, dynamic, hierarchical way such as to self-construct an autonomously working organization characterizable as a ‘whole’ or ‘operational unit’ in virtue of a boundary selectively separating it from its environment — a kind of universe unto itself. We can hold that view of living systems regardless of the nature of the components that self-construct it, but on Earth we recognize those components as matter in the form of atoms and molecules, importing, converting, storing, releasing free energy, and actuated by it.
The precise description of the organization of living things differs widely among species. Think of an ant and an anteater. We can, however, specify characteristics of the ‘kind’ of organization that all species share here on Earth. For one thing, we can say a living system’s complexity exceeds current human cognitive ability to comprehend it, even with the aid of a powerful computer exo-cortexes. Arguably, in the future that characteristic of the organization in living things may prove non-constitutive.
We can say also that the organizational state of living systems resembles that of a man-made machine, like a super-jet airplane or a super-computer, though not made by man and not obviously made for a purpose. We can think of a living system as a different ‘kind’ of machine than man-made machines. We can see that living machines exhibit a natural, or non-contrived ability to keep many of its internal variables constant, or within narrow bounds — it qualifies as a homeostasis machine.
A living system’s homeostatic ability plays a critical role in defining its uniqueness, as it enables it to homeostatically regulate the most important variable required for its continued living: an organization, whatever its description, that perpetuates its existence as a living system. Through the activity of its organization, the living system produces those components that provide the structural basis for the self-construction of its state as an autonomously working organization. If a living system cannot maintain its organization, it cannot produce the structure whose self-constructed coordinated interactions enable it to remain a living machine.
We can view a living system then as:
- a self-constructed machine organized as a network of interactions that produce, cyclically, the components whose self-organized interactions self-construct the system’s self-perpetuating network of interactions.
- a self-constructed machine organized as a network of interactions that can respond to perturbations either by self-correction of its disturbed organization (homeostasis), or by reorganizing itself into a different self-perpetuating network of interactions (adaptability; reproduction).
We can encapsulate that view of living systems preliminarily as ‘self-constructed self-perpetuating homeostatic machines’. Maturana and Varela[72] introduced the term ‘autopoiesis’ and ‘autopoietic organization’ to encapsulate that view of living machines as self-constructed self-perpetuating homeostatic machines as we have characterized them.</ref> Bitbol and Luisi expressed the definition of autopoiesis as follows:[74]
The theory of autopoiesis...captures the essence of cellular life by recognizing that life is a cyclic process that produces the components that in turn self-organize in the process itself, and all within a boundary of its own making.
That view of a living system reveals a special property of homeostasis in living machines: adaptability. A human, to take an example mammal, self-perpetuates a life-sustaining organization despite enormous perturbations of its organization during embryonic and fetal ‘development’. It does it by self-reorganizing — the homeostatic property of adaptability. If we think a fetus or a child an immature adult, we must think adults aged fetuses or children. As one individual or identity, fetus and adult represent a single self-constructing self-perpetuating homeostatically adaptable machine.
Ontogeny highlights the living system’s unique property of homeostasis in targeting with highest priority the maintenance of an organization that produces components that self-organize a network of interactions that perpetuates that organization — including its networks of interactions that retain its homeostatic property of adaptability. Homeostatic reorganization goes on continuously. The living machine maintains networks of interactions that define it as a self-constructing self-sustaining machine.
The self-constructed self-perpetuating homeostatic machine also produces its own boundary, as without that it could not maintain its organization against all the chaos outside.
A man-made, non-living machine yields products other than itself, products for human use. A living machine yields itself as its product, a product in continuous production, no matter how much it must modify itself in the process.
Therein defines the living machine’s autonomy —- it works in its own behalf to construct and sustain itself. So central to a living machine's uniqueness, its homeostatic organizational ability to produce components whose interactions self-organize a self-perpetuating organization, that before accumulated perturbations of its organization overwhelms its homeostatic ability, the machine self-reproduces.
By this view, neither growth nor reproduction necessarily constitute ‘primary’ abilities of living machines, as both occur, in life on Earth, as the consequence of the homeostatic adaptable activities of the self-constructing organization that produces components whose interactions realize that organization, along with its homeostatic adaptability. On other worlds, living systems need not necessarily grow or reproduce, so long as they can, in some way, construct the components that can self-organize to construct the organization that can produce those components, including the system’s own boundary whose character enables its individuality and access to resources and waste disposal.
Scientists can model and even synthesize experimental living machines that satisfy the basic criteria of a self-constructing self-perpetuating homeostatic machine (see[74]).
Access to resources alone cannot carry the day for a self-constructing homeostatic machine. It must have the ability, as part of its self-constructed organization, to recognize the resources it needs in order to sustain its organization. Recognition, however mediated, implies a type of ‘cognition’. In that case, for living machines to have an organization that produces the components that self-construct their-own component-producing organization, that organization must devote some of its activities to a type of cognition that enables it to recognize import resources and dispose of waste.
Those considerations dictate that a full description, or definition, of a living machine include the following:
- An organization of components capable of producing and reproducing, cyclically, the components that self-organize to construct the organization of components that produces those components;
- The components produced must self-construct a boundary between the machine and the environment, of a nature that enables the machine to trade with the environment, acquiring the materials and/or energy required to sustain its self-perpetuating organization;
- The components produced must self-construct an organization that has the cognitive ability to recognize the resources it needs to import and the wastes it needs to export.
- The components produced must self-construct an organization that has the homeostatic ability to ‘correct’/’accommodate’ perturbations of the organization, or to reorganize appropriately to sustain a self-perpetuating organization;[75]
With those conditions realized, we can then ask about the details of the mechanisms or conditions that effect that realization in Earth’s living machines, whose components are molecules that self-construct networks comprising an organization that recursively constructs its components of such nature that the organization they produce can operate autonomously with homeostatic adaptability to sustain or reorganize itself as a cognizing compartmented system capable of escaping thermodynamic equilibrium through repeated self-reproduction.
See also in Citizendium
- Origin of life
- Evolution
- Evolution of cells
- Biology
- Systems biology
- Metabolism
- The four Aristotelian causes of living things
- Homeostasis (Biology)
Appendix A
Living things share some very specific features not always explicitly stated above. For example,
- some evidence supports the proposition that all extant living things descended from a common ancestor [76] Other evidence suggests that “Extant life on Earth is descended not from one, but from three distinctly different cell types. However, the designs of the three have developed and matured, in a communal fashion, along with those of many other designs that along the way became extinct.” [77]
- only pre-existing cells can 'manufacture' new cells;
- only pre-existing multicellular organisms can manufacture new multicellular organisms;
- a membrane encloses every cell, protecting each from dissolution into its external environment;
- the cell membrane contains molecular systems that enables the cell to import usable matter and energy and to export unusable matter and energy, and others that enable it to send and receive signals to and from other cells;
- all cells and multicellular systems eventually die.
Appendix B
Selected definitions of life
Marcello Bárbieri, Professor of Morphology and Embryology at the University of Ferrara, Italy, collected an extensive list of definitions of “Life” from scientists and philosophers of the 19th and 20th centuries.[78] Those selected below resonate with the systems and thermodynamic perspectives of living systems:
- "The broadest and most complete definition of life will be "the continuous adjustment of internal to external relations". — Herbert Spencer (1884)
- "It is the particular manner of composition of the materials and processes, their spatial and temporal organisation which constitute what we call life." — A. Putter (1923)
- "A living organism is a system organised in a hierarchic order of many different parts, in which a great number of processes are so disposed that by means of their mutual relations, within wide limits with constant change of the materials and energies constituting the system, and also in spite of disturbances conditioned by external influences, the system ts generated or remains in the state characteristic of it, or these processes lead to the production of similar systems." — L. von Bertalanffy (1933)
- "Life seems to be an orderly and lawful behaviour of matter, not based exclusively on its tendency to go from order to disorder, but based partly on existing order that is kept up." — E. Schrodinger (1944)
- "Life is made of three basic elements: matter, energy and information. Any element in life that is not matter and energy can be reduced to information." — P. Fong (1973)
- "A living system is an open system that is self-replicating, self-regulating, and feeds on energy from the environment." — R. Sattler (1986)
Published collections of definitions of 'Life'
- Popa R (2004) Chronology of Definitions and Interpretations of Life. In: Popa R (ed.) Between Necessity and Probability: Searching for the Definition and Origin of Life. Berlin: Springer-Verlag 2004: pp 197-205 (Quotes and source-citations from 1885 to 2002)
- Barbieri M (2003) Appendix: Definitions of Life. In: The Organic Codes: An Introduction to Semantic Biology. Cambridge, UK: Cambridge University Press ISBN 0521824141 (Quotes from 1802 to 2002)
The gray zone
Not all entities that otherwise qualify as living reproduce themselves, although they exist as reproduced living things. Biologists call such living things 'sterile'. Examples include programmed sterility (e.g., worker ants, mules); acquired sterility (due to acquired injury (disease) to the reproductive process; access sterility (lack of reproductive fitness); voluntary sterility (e.g., human couples). Obviously living things with the capacity to reproduce may die before reaching the reproductive stage in their life-cycle. Conversely, non-reproducing individuals may still effect reproduction of copies of their genes by facilitating the reproduction of kin, who share many genes (see kin selection).
Viruses would not qualify strictly as living things, but manage to 'reproduce' in living systems.
One might ask whether a spermatozoon qualifies as a living entity. From the thermodynamic perspective, one might answer affirmatively, as it keeps itself ‘living’ by doing cellular work. It has a compartmentalized internal organization functioning to keep it far-from-equilibrium. In that respect it resembles a motile bacterium. A spermatozoon reproduces, but in a different way than a motile bacterium: it does it through its parent’s progeny, which the spermatozoon plays an essential role in generating. It doesn’t have to hijack a cell’s machinery to reproduce; it cooperates with another cell (an ovum) to generate cells with machinery to reproduce it. Moreover, in reproducing that way, it subjects itself to meiotic crossover variation, just as its parent’s progeny does, contributing to the variation needed by natural selection to perpetuate the process of living on an earth with ever-changing environments.
References
Citations and Notes
- ↑ Note: Some words, so-called ‘semantic primes’ have distinct meanings not definable in terms of other words. Ultimately, all definitions converge on about 70 semantic primes that occur universally among languages. Semantic primes include the verb ‘live’ but not the noun ‘life’. (Semantic Primes and Cultural Scripts in Language: Learning and Intercultural Communication.)
- Carol Cleland of NASA’s Astrobiology Institute suggests that scientists are not really interested in what the word 'life' happens to mean in our language. "What we really need to focus on is coming up with an adequately general theory of living systems, as opposed to a definition of 'life'."Carol Cleland on "What is Life?"
- ↑ De Duve C (2004) Life Evolving: Molecules, Mind, and Meaning. Oxford University Press. New York ISBN 0195156056
- ↑ from Robinson R (2007) Both barriers and trait complementarity govern pollination network structure. PLoS Biol 5(2): e54 doi:10.1371/journal.pbio.0050054
- ↑ 4.0 4.1 Tsytovich VN, Morfill GE, Fortov VE, Gusein-Zade NG, Klumov BA, Vladimirov SV (2007) [http://dx.doi.org/10.1088/1367-2630/9/8/263
From plasma crystals and helical structures towards inorganic living matter.] New J. Phys. 9: 263 [peer-reviewed open-source electronic journal]
- Note: From the Abstract: “Complex plasmas [a state of matter common in outer space consisting of a mass of charged particles] may naturally self-organize themselves into stable interacting helical structures that exhibit features normally attributed to organic living matter. The self-organization is based on non-trivial physical mechanisms of plasma interactions involving over-screening of plasma polarization. As a result, each helical string composed of solid microparticles is topologically and dynamically controlled by plasma fluxes leading to particle charging and over-screening, the latter providing attraction even among helical strings of the same charge sign. These interacting complex structures exhibit thermodynamic and evolutionary features thought to be peculiar only to living matter such as bifurcations that serve as `memory marks', self-duplication, metabolic rates in a thermodynamically open system, and non-Hamiltonian dynamics. We examine the salient features of this new complex `state of soft matter' in light of the autonomy, evolution, progenity and autopoiesis principles used to define life. It is concluded that complex self-organized plasma structures exhibit all the necessary properties to qualify them as candidates for inorganic living matter that may exist in space provided certain conditions allow them to evolve naturally."
- ↑ Valentine JW et al. (1994) Morphological complexity increase in metazoans. Paleobiology 20:131-42
- ↑ Woese CR et al. (1990) Towards a natural system of organisms: proposal for the domains archaea, bacteria, and eucarya. Proc Natl Acad Sci USA 87:4576-9
- ↑ Baluska F, Volkmann D, Barlow PW. (2004) Eukaryotic Cells and their Cell Bodies: Cell Theory Revised. Ann Bot 94:9-32 Link to Full-Text
- Note: “…those who are aware of the most recent advances in plant cell biology…are convinced that Cell Theory, as it now stands, is absolutely incompatible with a cell-based organization of higher plants…and requires an update..Indeed, formulation of organismal theory of plant development, in which it is stated that it is not the cell but the whole multicellular organism that is the primary unit of plant life..has precipitated a crisis for Cell Theory as applied to plants. A consequence of the fact that the cytoplasms of plant cells are interconnected via plasmodesmata is that the individuality of the cell is given up in favour of an integrated and corporate cytoplasm that benefits the whole organism. This supracellular, or organismal, approach towards multicellularity seems to have allowed sessile plants to adapt to life on land and to evolve even within hostile environments.
- ↑ Note: Other boundaries of living systems include bark, shells, cell walls, skin, fur, and structures of the physical environment.
- ↑ How Many Genes Are in the Human Genome? at the Human Genome Project Information website hosted by the U.S. Department of Energy
- ↑ (a) The UniProtKB/Swiss-Prot Human Proteome Initiative [1]; (b) Norregaard Jensen O. (2004) Modification-specific proteomics: characterization of post-translational modifications by mass spectrometry. Current Opinion in Chemical Biology 8:33-41
- ↑ Valentine JW et al. (1994) Morphological complexity increase in metazoans. Paleobiology 20:131-42
Note: See the article, Cell, for a more thorough discussion of 'cell types'. - ↑ Andrea Falcon (2006) Aristotle on Causality
- ↑ Bothwell JHF. (2006) The long past of systems biology. New Phytologist 170:6-10 Link to Full-Text.
Note: We might interpret Aristotle's four components of 'causality' as four components of 'explanation', for as Bothwell writes: “Aristotle (384-322 BC) wanted to search for explanations of natural events that inspire wonder. His search led him to conclude that any question which might be asked about the behaviour of a complex, apparently designed, system might be answered if we knew four properties of that system. He called these the aitiai, a word which is usually rendered into English as 'causes', but which may be better translated as 'explanations' (Aristotle, APst 90a7-94b34; CA 715a1-17 [Aristotle. APst (Posterior Analytics), Trans: H. Tredennick (1960). Harvard University Press, Loeb Classical Library. (ISBN 0-674-99430-2)]).” - ↑ Note: For example, physical chemists cannot predict the properties of water from knowledge of its components, hydrogen and oxygen. The way hydrogen and water interact to form H2O, and the way H2O molecules interact, enables the properties of water to 'emerge'.
- ↑ Note: Following up on the example of water, the properties of its environment (e.g., temperature, pressure) affect the way the H2O molecules organize themselves, as ice, or liquid, or steam.
- ↑ Note: In relation to downward causation, the environment’s effect can sometimes reach down to the genetic recipe with molecular signals, altering the recipe’s expression and consequently the characteristics of the cells — so-called 'epigenetic' effects. When epigenetic alterations of gene expression occur in the reproductive organs, the system changes can be transmitted to the next generation. See
- Jablonka E, Lamb MJ (2005) Evolution in Four Dimension: Genetic, Epigenetic, Behavioral, and Symbolic Variation in the History of Life. Cambridge: MIT Press
- Gorelick R (2004) Neo-Lamarckian medicine. Med Hypotheses 62:299-303 PMID 14962644
- ↑ Walsh DM (2006) Organisms as natural purposes: the contemporary evolutionary perspective. Stud Hist Philos Biol Biomed Sci 37: 771-91
- ↑ Nobel D. (2006) The Music of Life: Biology Beyond the Genome. Oxford University Press, New York. ISBN 978-0-19-929573-9 Brief Biography Multiple Chapter Excerpts Online
- ↑ 19.0 19.1 Darwin C (1982; originally 1859) The Origin of Species By Means of Natural Selection or the Preservation of Favoured Races in the Struggle for Life. London: Penguin Books ISBN 9780140432053
- ↑ Note: A random pattern of parts has no order (it has maximum entropy) and no information. A living system has order in its organized state; it has computationally-rich informational content, and low entropy.
- ↑ Note: At birth — the big bang — the Universe received the gift of an energy account. The amount of energy received remains forever constant, though the energy can distribute itself into many smaller accounts. If and when it completely disperses itself throughout the Universe in the form of heat — energy's most degraded and least useful form — dispersing into many tiny accounts, with little or no difference in energy content among the accounts, it has degraded to the point it can no longer do work because no energy gradients (slopes) exist down which energy can flow to perform any work. At that point, when the Universe has reached a state of absent energy gradients, it reaches a state of equilibrium, one characterized by a completely random distribution of the initial energy account, diluted in the vastness of the expanding Universe.
- ↑ 22.0 22.1 Schneider ED, Sagan D (2005) Into the Cool: Energy Flow, Thermodynamics, and Life. Chicago: The University of Chicago Press. ISBN 0-226-73937-6 Chapter Excerpts and Reviews
- ↑ Note: Unless contrived to do work, heat has a disordering, entropy-increasing effect. The more heat put into a system at a given temperature, the greater the entropy. At lower temperatures, the same heat input can increase entropy more, because the greater degree of order present at lower temperatures means a greater fractional increase in disorder for the same heat input.
- ↑ Prigogine I, Stengers I (1997) The End of Certainty: Time, Chaos, and the New Laws of Nature. Free Press, New York. ISBN 0684837056
- ↑ Swenson R, Turvey MT (1991) Thermodynamic reasons for perception-action cycles. Ecol Psychol 3:317-48. Swenson R (1997) Thermodynamics, Evolution, and Behavior. In The Encyclopedia of Comparative Psychology G. Greenberg and M. Haraway (Eds), New York: Garland Publishers, Inc.
- ↑ Schneider ED, Kay JJ (1994) Life as a manifestation of the second law of thermodynamics. Math Computer Modelling 19:25-48
- ↑ Note: If an energy gradient promotes the origin of living systems because as the energy flows through them they accelerate the rate of entropy production of the Universe as a whole by exporting more entropy to their surroundings than they reduce their internal entropy, then the energy gradient might also promote the development of complexity in living systems, as complexity further reduces a living system’s internal entropy (greater organization). With sufficient organization, living systems can attain a degree of intelligence enabling them to fabricate energy-utilizing artifacts, like electrical lighting systems, fossil-fuel-based transportation systems, and solar and geothermal energy conversion to work. Those artifacts then would further contribute to dissipation of the life-promoting energy gradient and further accelerate the rate of entropy production of the Universe as a whole. Such considerations make it seem likely, most speculatively, that Nature’s abhorrence of energy gradients throughout the Universe would promote the origin and complexity development of living systems wherever physico-chemical circumstances permit, as a means of maximizing the rate of entropy production of the Universe as a whole.
- ↑ Note: ....or the progeny of some conspecific living systems. Many living systems coexist with similar living systems, constituting a 'species', or group of 'conspecifics'.
- ↑ Jablonka E, Lamb MJ (2005) Evolution in Four Dimension: Genetic, Epigenetic, Behavioral, and Symbolic Variation in the History of Life. Cambridge: The MIT Press
- ↑ Smolin L (1997) The Life of the Cosmos. New York: Oxford University Press. ISBN 019510837X
- ↑ Schulze-Makuch D, Irwin LN, Definition of Life. In Life in the Universe. Berlin: Springer-Verlag 2004: Chapter 2. pp 8-34 Link to Summary and Full-Text
- ↑ Lehn JM. (2002) Toward complex matter: supramolecular chemistry and self-organization. Proc Natl Acad Sci USA 99:4763-4768 PMID 11929970
- ↑ 33.0 33.1 Lehn JM (2002) Toward self-organization and complex matter. Science 295:2400-2403 [2]
- ↑ Note: The qualifier "in part" reflects the need to invoke not only supramolecular self-assembly but also evolutionary mechanisms of producing and selecting genes that yield proteins whose chemical properties enable interactions that tend to optimize functional self-organization — in other words, adaption. One must also invoke local real-time selective processes that confer stability and appropriate functionality to self-assembly, called homeostasis. Self-organization and adaptability conjoin to yield function.
- Heylighen F (2001) The Science of Self-organization and Adaptivity. In: Kiel LD (ed.) Knowledge Management, Organizational Intelligence and Learning, and Complexity: The Encyclopedia of Life Support Systems (EOLSS) Oxford: Eolss
- ↑ Reinhoudt DN, Crego-Calama M (2002) Synthesis beyond the molecule. Science 295:2403-2407 [3].
- ↑ Percec V, Ungar G, Peterca M (2006) CHEMISTRY: Self-assembly in action. Science 313:55-56 [4]
- ↑ Lehn JM. (2007) From supramolecular chemistry towards constitutional dynamic chemistry and adaptive chemistry. Chem Soc Rev 36:151-160 PMID 17264919
- ↑ Harold FM. (2005) Molecules into cells: specifying spatial architecture. Microbiol Mol Biol Rev 69:544-64 PMID 16339735
- ↑ Loewenstein WR. (1999) The Touchstone of Life: Molecular Information, Cell Communication, and the Foundations of Life. Oxford University Press, New York. ISBN 0-19-514057-5
- ↑ Noble D (2002) Modeling the heart—from genes to cells to the whole organ. Science 295:1678-82
- ↑ Sidney Brenner’s Nobel lecture (2002) “Nature’s Gift to Science”
- ↑ Brenner S (1998) Biological computation. Novartis Found Symp 213:106-11 PMID 9653718
- ↑ Dawkins R (1988) The Blind Watchmaker: Why the Evidence of Evolution Reveals a Universe Without Design. New York: W.W. Norton & Company, Inc. ISBN 0393304485 Excerpt from Amazon.com review: “The title of this 1986 work, Dawkins's second book, refers to the Rev. William Paley's 1802 work, Natural Theology, which argued that, just as finding a watch would lead you to conclude that a watchmaker must exist, the complexity of living organisms proves that a Creator exists. Not so, says Dawkins: "the only watchmaker in nature is the blind forces of physics, albeit deployed in a very special way... it is the blind watchmaker." (Physics, of course, includes open-system non-equilibrium thermodynamics, pivotal to understanding how living systems sustain themselves.)
- ↑ 44.0 44.1 44.2 Kauffman S (2003) Molecular autonomous agents. Philos Transact A Math Phys Eng Sci. 361:1089-99 PMI: 12816601
- ↑ Kauffman SA (2000) Investigations. Oxford University Press, Oxford. ISBN 019512104X Publisher’s description and reviews
- ↑ Franklin S, Graesser A (1996) Is it an Agent, or just a Program?: A Taxonomy for Autonomous Agents. Proc Third Int Workshop on Agent Theories, Architectures, and Languages, Springer-Verlag
- ↑ Kauffman S (2003) The Adjacent Possible
- ↑ 48.0 48.1 Barabási AL (2002) Linked: The New Science of Networks. Cambridge, Mass: Perseus Pub. ISBN 0-7382-0667-9
- ↑ Watts DJ. (2007) A twenty-first century science. Nature 2007;445:489
- ↑ Alon U (2003) Biological networks: the tinkerer as an engineer. Science 301: 1866-7 PMID 14512615
- ↑ Hartwell LH, Hopfield JJ, Leibler S, Murray AW. (1999) From molecular to modular cell biology. Nature 402:C47-C52 PMID 10591225
- ↑ Darwin C. (1859) On the origin of species by means of natural selection, or the preservation of favoured races in the struggle for life. 1st edition. Chap. VI, p190 “Difficulties on Theory”
- Darwin wrote: “The illustration of the swimbladder in fishes is a good one, because it shows us clearly the highly important fact that an organ originally constructed for one purpose, namely flotation, may be converted into one for a wholly different purpose, namely respiration.”
- Stephen Jay Gould and others dispute Darwin on the direction of exaptation between swimbladder and lung, though not the rality of exaptation: Gould writes: “Darwin was wrong; ancestral vertebrates had lungs… The first vertebrates maintained a dual system for respiration: gills for extracting gases from seawater and lungs for gulping air at the surface. A few modern fishes, including the coelacanth, the African bichir Polypterus, and three genera of lungfishes, retained lungs… In two major lineages of derived bony fishes — the chondrosteans and the teleosts -- lungs evolved to swim bladders by atrophy of vascular tissue to create a more or less empty sac and, in some cases, by loss of the connecting tube to the esophagus (called the trachea in humans and other creatures with lungs). See: Gould SJ. (1993) Eight Little Piggies: Reflections in Natural History. Norton, New York. ISBN 039303416X.
- ↑ See definition of ‘exapt’
- ↑ Lenski RE, Barrick JE, Ofria C. (2006) Balancing Robustness and Evolvability. PLoS Biology 4(12): e428
- ↑ 55.0 55.1 Alon U (2007) Simplicity in biology. Nature 446:497
- ↑ Prill RJ et al. (2004) Dynamic properties of network motifs contribute to biological network organization. PLoS Biol 3: e343
- ↑ Sporns O, Kotter R (2004) Motifs in brain networks. PLoS Biol 2: e369
- ↑ Alon U (2007) An Introduction to Systems Biology: Design Principles of Biological Circuits. Boca Raton: Chapman and Hall/CRC
- ↑ Note: This article takes the view that a cell constitutes the unit ‘living systems’, and that cellular subsystems, like transcription networks and metabolic pathways, qualify as ‘biological systems’ but not as ‘living systems’.
- ↑ Note: That does not explain how living systems came to acquire the ability to utilize available energy in the first place. To explain that requires knowledge of the origin of living systems. See Origin of life
- ↑ Note: Usable energy, also called ‘free energy’, in virtue of its organized state that flows downhill to dissipated uselessness (disorder), has all the attributes of information.
- ↑ Richardson PJ, Boyd R. (2006) Not By Genes Alone. The University of Chicago Press, Chicago. ISBN 0-226-71212-5
- ↑ Goodwin B. (1994) How The Leopard Changed Its Spots: The Evolution Of Complexity. Princeton University Press, Princeton. ISBN 0-691-08809-8
- ↑ Deacon TW (1997) The Symbolic Species: The Co-Evolution of Language and the Brain. New York: W.W. Norton & Company, Inc. ISBN 0393038386
- ↑ Danchin A et al. (2007) The extant core bacterial proteome is an archive of the origin of life. Proteomics 7:875–89
- ↑ Hood L (2003) Systems biology: integrating technology, biology, and computation. Mechanisms of Ageing and Development 124:9-16
- ↑ Bains W (2004) Many chemistries could be used to build living systems. Astrobiology PMID 15253836 4:137-67
- ↑ Ball P. (2005) Water and life: Seeking the solution. Nature 436:1084-1085 Link to Full-Text
- ↑ Note: We can arrive at a more-or-less empirically sound explanation of what constitutes living systems without having a good explanation for how they arose in the first place, because we can study the here-and-now and not the there-and-then.
- ↑ Odling-Smee FJ, Laland KN, Feldman MW (2003) Niche Construction; The Neglected Process in Evolution. Princeton: Princeton University Press. ISBN 0691044384
- ↑ Damasio A. (2003) Looking for Spinoza: Joy, Sorrow, and the Feeling Brain. Orlando, Florida: Harcourt, Inc. ISBN 978-0099421832, ISBN 978-0151005574
- ↑ 72.0 72.1 Maturana HR, Varela FJ. (1973) Autopoiesis: The Origin of the Living. In: Autopoiesis and Cognition: The Realization of the Living. D. Reidel Publishing Company, Dordrect:Holland. ISBN 90-277-1015-5
- ↑ Hofmeyr JH (2007) The biochemical factory that autonomously fabricates itself: A systems biological view of the living cell. In: Boogerd FC, Bruggeman FJ, Hofmeyr JH, and Westerhoff HV (editors). Systems Biology: Philosophical Foundations. Elsevier, Amsterdam. ISBN 13:978-0-444-52085-2 (see page 225)
- ↑ 74.0 74.1 Bitbol M, Luisi PL. (2004) Autopoiesis with or without cognition: defining life at its edge. PMID 16849156
- ↑ Note: Taking homeostasis to mean stability of self-construction and self-sustenance, a living machine might achieve that by incorporating the perturbation (e.g., a foreign molecule) ito its organization.
- ↑ Kurland CG, Collins LJ, Penny D (2006) Genomics and the irreducible nature of eukaryote cells. Science 312: 1011-14
- ↑ Woese CR (2002) On the evolution of cells. Proc Natl Acad Sci U S A 99:8742-7 PMID 12077305
- ↑ Bárbieri M. (2003) The Organic Codes; An Introduction to Semantic Biology. Cambridge: Cambridge University Press.