Macromolecular chemistry: Difference between revisions
imported>John Stephenson m (moved Macromolecular chemistry/Draft to Macromolecular chemistry: citable version policy) |
Pat Palmer (talk | contribs) mNo edit summary |
||
(3 intermediate revisions by 3 users not shown) | |||
Line 9: | Line 9: | ||
In industry, the value of synthetic macromolecules as plastics and nylon, has risen enormously over the last 60 years. They have made it possible to mould shapes that would have been impossible to create without them. When they were first developed, their resistance to rupture and degradation was seen as a profound advantage, but nowadays we seek more biologically degradable plastics such as polyethyleneglycol that pollute the environment less. | In industry, the value of synthetic macromolecules as plastics and nylon, has risen enormously over the last 60 years. They have made it possible to mould shapes that would have been impossible to create without them. When they were first developed, their resistance to rupture and degradation was seen as a profound advantage, but nowadays we seek more biologically degradable plastics such as polyethyleneglycol that pollute the environment less. | ||
Biological macromolecules include, besides the molecules already mentioned, [[enzyme]]s, and [[polysaccharide]]s, such as cellulose and starch. The better understanding of the basic behavior of polymer molecules has enhanced our knowledge of these biological molecules, and studies of partially charged [[polyelectrolytes]] have led to a deeper insight into their biological function. The investigations of the three-dimensional structure of macromolecules, (their [[configuration]] and [[conformation]]), have led to the identification of specific regions that perform specialized activities. A good example is the catalytic role of particular amino acid residues in polypeptide enzymes and the role of [[functional group]]s such as [[biotin]] or [[riboflavin]] in cellular metabolism. The folding of macromolecules is now a topic of much scientific investigation, since the correct folding of these polymers is a critical factor for normal function. Abnormal folding of particular proteins is the cause of several diseases, including [[Alzheimer disease|Alzheimer's]] and [[Creutzfeldt-Jakob disease]] (CJD) . | Biological macromolecules include, besides the molecules already mentioned, [[enzyme]]s, and [[polysaccharide]]s, such as cellulose and starch. The better understanding of the basic behavior of polymer molecules has enhanced our knowledge of these biological molecules, and studies of partially charged [[polyelectrolytes]] have led to a deeper insight into their biological function. The investigations of the three-dimensional structure of macromolecules, (their [[configuration]] and [[conformation]]), have led to the identification of specific regions that perform specialized activities. A good example is the catalytic role of particular amino acid residues in polypeptide enzymes and the role of [[functional group]]s such as [[biotin]] or [[riboflavin]] in cellular metabolism. The folding of macromolecules is now a topic of much scientific investigation, since the correct folding of these polymers is a critical factor for normal function. Abnormal folding of particular proteins is the cause of several diseases, including [[Alzheimer's disease|Alzheimer's]] and [[Creutzfeldt-Jakob disease]] (CJD) . | ||
==Macromolecules== | ==Macromolecules== | ||
Line 69: | Line 69: | ||
== Introduction to Physical polymer chemistry== | == Introduction to Physical polymer chemistry== | ||
: ''The subtopics of '''[[Physical polymer chemistry]]''' are [[Statistical physics]], [[Statistical thermodynamics]], [[Graph theory]], [[Group Theory]], [[Monte Carlo simulations]], [[Scaling theory]], [[Physics of polymer solutions]], [[Stochastic | : ''The subtopics of '''[[Physical polymer chemistry]]''' are [[Statistical physics]], [[Statistical thermodynamics]], [[Graph theory]], [[Group Theory]], [[Monte Carlo simulations]], [[Scaling theory]], [[Physics of polymer solutions]], [[Stochastic process]]es and [[Chemistry of polymer solutions]].'' | ||
The physical properties of a macromolecule depend largely upon its composition. A simple hydrocarbon chain has a 'skeleton' of [[Carbon|carbon]] atoms to which [[Hydrogen|hydrogen]] atoms are attached; the general composition of a pure hydrocarbon is C<sub>n</sub>H<sub>2n+2</sub>, where all bonds between the carbon atoms have been completely saturated (by hydrogen). The simplest hydrocarbon is [[methane]] (CH<sub>4</sub>); methane is a [[greenhouse gas]], and is highly inflammable and explosive. The next largest hydrocarbons, ethane, propane and butane, are also gases at room temperature (20˚C), and propane and butane are widely used as fuels for heating. As can be deduced from their composition, hydrocarbons with longer chains than butane tend to be liquid at room temperature, and very high chain length hydrocarbons are solids. | The physical properties of a macromolecule depend largely upon its composition. A simple hydrocarbon chain has a 'skeleton' of [[Carbon|carbon]] atoms to which [[Hydrogen|hydrogen]] atoms are attached; the general composition of a pure hydrocarbon is C<sub>n</sub>H<sub>2n+2</sub>, where all bonds between the carbon atoms have been completely saturated (by hydrogen). The simplest hydrocarbon is [[methane]] (CH<sub>4</sub>); methane is a [[greenhouse gas]], and is highly inflammable and explosive. The next largest hydrocarbons, ethane, propane and butane, are also gases at room temperature (20˚C), and propane and butane are widely used as fuels for heating. As can be deduced from their composition, hydrocarbons with longer chains than butane tend to be liquid at room temperature, and very high chain length hydrocarbons are solids. | ||
Line 79: | Line 79: | ||
Macromolecules do exhibit different behaviour as well. Physical chemists use light diffraction (direct and indirect - or first- and second-order light diffraction) to determine the shape of a molecule, just as a rotating cigar will diffract light differently to a rotating ball. Shape can be a clue to the functionality of a molecule in vivo. Similar results can be obtained from viscosimetric observations, where the shape of a molecule in terms of its hydrodynamic radius can be determined by comparing the behavior of the solvent to that of the solution. These results can be calculated from equations given in some of the textbooks below. | Macromolecules do exhibit different behaviour as well. Physical chemists use light diffraction (direct and indirect - or first- and second-order light diffraction) to determine the shape of a molecule, just as a rotating cigar will diffract light differently to a rotating ball. Shape can be a clue to the functionality of a molecule in vivo. Similar results can be obtained from viscosimetric observations, where the shape of a molecule in terms of its hydrodynamic radius can be determined by comparing the behavior of the solvent to that of the solution. These results can be calculated from equations given in some of the textbooks below. | ||
Another way to describe the behavior of macromolecules is by using [[Stochastic | Another way to describe the behavior of macromolecules is by using [[Stochastic process|stochastics]] as the mathematical description of observed behavior. The conformation of a macromolecule in a solvent can be described as a stochastic process with the configuration as the leading part and the electric behavior (e.g. amount of charges, occurrences of hydrogen bonds, the nature of the interactions between the solute and the solvent) as a series of smaller, fast fluctuating terms as a function of time. This description leads the way to numerical analyses, and is one of the ways used in [[Monte Carlo simulations]]. The numerical answer can be used to help explain some of the hydrodynamic aspects and behavior of the macromolecule. | ||
=== Unsaturated hydrocarbons === | === Unsaturated hydrocarbons === | ||
Line 108: | Line 108: | ||
By contrast, the '''conformation''' of a macromolecule is its (chemical) behavior in the environment in which it is observed. Often, that behavior is determined by the configuration of the molecule as changed by specific components, either in the molecule or in its environment, that affect its structure, behavior and responses. For example, the ''configuration'' of a DNA molecule is of a double-stranded ''α''-helix. By contrast, its ''conformation'', when not in a particular active mode, is that of an imploded, compact, super-coiled molecule, nearly oblivious to its environment, until it receives an awakening 'call'. | By contrast, the '''conformation''' of a macromolecule is its (chemical) behavior in the environment in which it is observed. Often, that behavior is determined by the configuration of the molecule as changed by specific components, either in the molecule or in its environment, that affect its structure, behavior and responses. For example, the ''configuration'' of a DNA molecule is of a double-stranded ''α''-helix. By contrast, its ''conformation'', when not in a particular active mode, is that of an imploded, compact, super-coiled molecule, nearly oblivious to its environment, until it receives an awakening 'call'. | ||
This same difference between configuration and conformation can be seen in many macromolecules. Some behave as if they 'remember' their old shape, returning to it when put under 'a stress'. Partially charged polyelectrolytes form a loosely-wound knot (like a very loosely-wound watery knot of wool), fluctuating in density and configuration during its normal state as being the solute. Many polyelectrolytes bond very strongly to metal ions, generally producing tightly bound coils with the metal ion in its middle and hydrophilic groups exposed to the exterior, most likely a watery solution. When combined with another solution containing metals, they tend to form 'cages'—sometimes very tightly bound cages—around these metal-ions, that precipitate during the reaction. Similar properties for poly(acrylic) acid (PAA), that can selectively bind heavy metals, are exploited for environmental cleanup. After treating silt the precipitate is rich in heavy (poisonous) metals, such as [[Cadmium|Cd]], [[ | This same difference between configuration and conformation can be seen in many macromolecules. Some behave as if they 'remember' their old shape, returning to it when put under 'a stress'. Partially charged polyelectrolytes form a loosely-wound knot (like a very loosely-wound watery knot of wool), fluctuating in density and configuration during its normal state as being the solute. Many polyelectrolytes bond very strongly to metal ions, generally producing tightly bound coils with the metal ion in its middle and hydrophilic groups exposed to the exterior, most likely a watery solution. When combined with another solution containing metals, they tend to form 'cages'—sometimes very tightly bound cages—around these metal-ions, that precipitate during the reaction. Similar properties for poly(acrylic) acid (PAA), that can selectively bind heavy metals, are exploited for environmental cleanup. After treating silt the precipitate is rich in heavy (poisonous) metals, such as [[Cadmium|Cd]], [[Copper|Cu]] and other toxic metals such as [[Lead|Pb]] and [[Mercury|Hg]], either in their metallic form or as tightly bound salt. | ||
Biological catalysts or [[Enzymes|enzymes]] show the same behavior as other catalysts, except that most biological catalysts need a particular configuration or folding in three dimensions to be effective. Even slight aberrations from this configuration can dramatically affect their activity. Many proteins even exploit these conformation changes to switch between 'on' and 'off' states. For example, in the brain, signals are carried by a wide variety of molecules that interact with polypeptide receptors on the surface of neuronal cells. The receptors are macromolecules that span the cell membrane; on the outside of the cell, the receptor forms a 'pocket' that binds specific signaling molecules and this initiates a change in the receptors conformation. This change can propagate across the membrane and affect the function of the receptor molecule inside the cell, often starting a signaling cascade. Compare it to activating an alarm system using a central key, it affects all areas controlled by that alarm system—signaling those parts of importance with the needed and appropriate information. | Biological catalysts or [[Enzymes|enzymes]] show the same behavior as other catalysts, except that most biological catalysts need a particular configuration or folding in three dimensions to be effective. Even slight aberrations from this configuration can dramatically affect their activity. Many proteins even exploit these conformation changes to switch between 'on' and 'off' states. For example, in the brain, signals are carried by a wide variety of molecules that interact with polypeptide receptors on the surface of neuronal cells. The receptors are macromolecules that span the cell membrane; on the outside of the cell, the receptor forms a 'pocket' that binds specific signaling molecules and this initiates a change in the receptors conformation. This change can propagate across the membrane and affect the function of the receptor molecule inside the cell, often starting a signaling cascade. Compare it to activating an alarm system using a central key, it affects all areas controlled by that alarm system—signaling those parts of importance with the needed and appropriate information. | ||
Line 119: | Line 119: | ||
==References== | ==References== | ||
<div class="references-small" style="-moz-column-count:2; -webkit-column-count:2; column-count:2;"> | <div class="references-small" style="-moz-column-count:2; -webkit-column-count:2; column-count:2;"> | ||
{{reflist}} | |||
</div> | </div>[[Category:Suggestion Bot Tag]] |
Latest revision as of 10:29, 19 December 2024

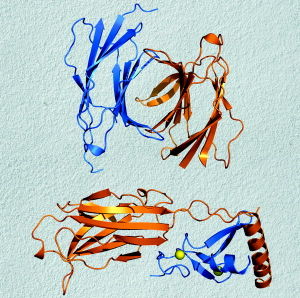
Macromolecular chemistry (from macro = large) is the study of the physical, biological and chemical structure, properties, composition, and reaction mechanisms of macromolecules. A macromolecule is a molecule that consists of one or more types of repeated 'building blocks'. The building blocks are called monomeric units (monomers).
Macromolecules (also known as polymer molecules) appear in daily life in the form of plastic, styrofoam, nylon, etc. These polymers, i.e., substances consisting of polymer molecules, are of great technological importance and are used in the manufacturing of all sorts of goods, from automobile parts to household appliances. The artificial polymer molecules usually exist of long repetitions of identical monomers, either in chains or networks.
In molecular biology macromolecules (biopolymers) play a very important role: the well-known molecules DNA, RNA, and polypeptides (proteins) are examples of macromolecules. In molecular biology one is mostly interested in macromolecules in solution, usually dissolved in water. The biological function of macromolecules in living cells is a highly relevant and widely studied topic of research. Although, strictly speaking, biopolymers belong to the class of polymer molecules, there is a tendency not to use the latter name in biological applications, but to speak of macromolecules. The term "polymer" is usually reserved for the substances (plastic, nylon, etc.) manufactured in bulk by the chemical industry.
In industry, the value of synthetic macromolecules as plastics and nylon, has risen enormously over the last 60 years. They have made it possible to mould shapes that would have been impossible to create without them. When they were first developed, their resistance to rupture and degradation was seen as a profound advantage, but nowadays we seek more biologically degradable plastics such as polyethyleneglycol that pollute the environment less.
Biological macromolecules include, besides the molecules already mentioned, enzymes, and polysaccharides, such as cellulose and starch. The better understanding of the basic behavior of polymer molecules has enhanced our knowledge of these biological molecules, and studies of partially charged polyelectrolytes have led to a deeper insight into their biological function. The investigations of the three-dimensional structure of macromolecules, (their configuration and conformation), have led to the identification of specific regions that perform specialized activities. A good example is the catalytic role of particular amino acid residues in polypeptide enzymes and the role of functional groups such as biotin or riboflavin in cellular metabolism. The folding of macromolecules is now a topic of much scientific investigation, since the correct folding of these polymers is a critical factor for normal function. Abnormal folding of particular proteins is the cause of several diseases, including Alzheimer's and Creutzfeldt-Jakob disease (CJD) .
Macromolecules
Macromolecules have a molecular mass larger than most simple molecules; in general, they have a mass of more than 1000 Dalton (or unified atomic mass unit), but the molecular weight of some can be as large as millions of Daltons (even billions in the case of DNA). Their mass and size often leads to them breaking up into smaller pieces simply due to the shear resistance experienced by the macromolecule in a solvent. Any macromolecule, staying in a simple unfolded stretched filament configuration, is experiencing stress shear due to its length and overall mass. For that reason macromolecules have a practical way to avoid these excess forces by folding. Some macromolecules in a solvent fold into an alpha helix shape, some in more complex folding structures, but all according to the possible biological functions that these molecules should exert when in a cellular environment. Complex molecules like DNA, RNA and many proteins also use coiling and super-coiling to regain a functional three dimensional conformation. Often these long molecules hide their basic backbones, but ensure stability and functionality—in essence their own existence and safety. In the laboratory artificial macromolecules are used with a mean molecular mass up to 5 million Dalton with a small spread in the weight—to have a sample suitable for research. (Long and heavy molecules tend to get disrupted, leading to smaller chains that can influence the measurements done on these molecules, and make it hard to understand results in an unambiguous way. Because of these shear breaks the average molecular weight of a macromolecular sample will have a large deviation around the mean value.)
The mechanism of supercoiling and folding is presently being researched in detail, as its biological function, and failure therein, has reportedly been connected to diseases like Alzheimer's and Creutzfeldt-Jakob disease (CJD). Large biological molecules seem to use another mechanism similar to the so-called Khohlov-implosion of macromolecules, a kind of implosive diminishing of the size of the dissolved molecule where its hydrodynamic radius diminishes by a factor of 1000 or more. This can be visualized by the super-coiled structure of DNA when inactive. DNA then has the same cigar resulting shape and consists of the total of all of the molecule in one chromosome comprised in a very compact way. This implosion seems to be based upon specific environmental conditions based upon charge effects and low concentrations of (possibly) interfering other kinds of macromolecules.
Economics and environment
Substances made from macromolecules can have very unusual properties that can be exploited in a wide range of applications. Importantly, plastics and other polymeric substances contribute substantially to the global economy. Whole industries are based on the use of plastics, including the packaging industries, toy manufacturers, automotive industry and household appliances. The downside, however, is the resistance of plastics to chemical degradation, causing them to persist in the environment and the food chain. Plastic bags lead to the death of many animals, be it by animals swallowing the plastic directly, or, later in the food chain, by predators eating plastic-containing prey. New artificial materials have been produced that are more easily part of chains of decay and composting. The PET-bottle is an example of these new materials. PET (polyethylene terephthalate) is a thermoplastic polymer resin of the polyester family that is used for making synthetic fibers as well as drink containers. PET is fully recyclable, as two depolymerisation methods - methanolysis and glycolosis - can be used to reduce PET to either a monomer or the original raw materials.[1]
Sources of raw material
Natural polymers do occur. The most familiar is natural rubber, also known as latex. It has been used for the production of tires (after vulcanization), paint, and even by the clothing industries. Natural rubber is an elastic and has been artificially created by polymerization of isoprene. A byproduct of the research into these industrial rubbers was Silly Putty. Most (artificial) plastics are made from refined crude oil. Because they are hard to produce otherwise, their continued availability is tied to petroleum security. However, some newer biological process, such as polylactide production, and Dupont's biological polyethylene glycol monomer synthesis route, use biological feed-stocks instead.[2]
Simple polymers
Simple polymers are the products of polymerization reactions. Polymerization reactions are known for their exothermic nature (their ability to release energy in the form of heat) and their fast kinetics. They continue until one of the reactants has been used, as for instance in the formation of polyurethane. As demonstrated in many high school classes, adding small amounts of ethene and urea to an acidic solution of water results in a high volume polymer in a seemingly never ending process. A very well known biological polymer is starch. Starch is a complex carbohydrate that contains two molecules: amylose and amylopectin, both of which are large polymers of glucose. Starch is soluble in water, and is used as a way of storing excess glucose by plants; it is mainly found in their fruit, seed rhizomes or tubers. Starch also plays a role in storing energy in the bodies of animals, although the two kinds of starch differ in composition. Some artificial polymers exhibit behavior not seen in other materials; for example, kevlar is a composite material that is stronger than steel but which weighs very little in comparison and is very flexible. Such materials have found many uses, from bullet-proof vests to enforcing more rigidity in the hull of aeroplanes. Another polymer of importance is silicone, famous by now for its application in the building industry as well as in medicine, reconstructive surgery as well as cosmetic surgery. Silicone is a polymer with a repetitive siloxane group as the backbone.
The behavior of a large group of polymers can be divided in two groups, the thermoplasts and the thermoharders. Thermoplasts respond to heat by becoming more fluid in their behavior, enabling them to be molded to any desired shape. This behavior can also be achieved by adding substances to the polymer (or plastic) called weakeners. Thermoharders are characterized by responding with a more crystalline structure upon heating, ultimately creating a granular resulting material. Many composite materials use these two behaviors in making plastics for special purposes. The thermoplastic behavior of polymers used for clothing, nylon, polyester makes them easier to wash and handle, but also introduces a danger. When heated extendedly they turn into a very hot liquid state, some to the point of burning. If they catch fire while being worn, severe injuries can occur as the materials melt get very sticky and burn into the skin.
(Poly)peptide polymers
One of the first artificial polypeptides made was nylon. Most nylons are condensation copolymers formed by reacting equal parts of a diamine and a dicarboxylic acid, so that amide bonds form at both ends of each monomer in a process analogous to polypeptide biopolymers. Because the reaction also produces H2O the reaction is called a condensation. The most common variant is nylon 6-6, which refers to the fact that the diamine (hexamethylene diamine) and the diacid (adipic acid) each donate six carbons to the polymer chain (the numerical suffix specifies the numbers of carbons donated by the monomers; the diamine first and the diacid second). As with other regular copolymers, like polyesters and polyurethanes, the 'repeating unit' consists of one of each monomer, so that they alternate in the chain. As each monomer has the same reactive group on both ends, the direction of the amide bond reverses between each monomer, unlike natural polyamide proteins which have overall directionality: C terminal → N terminal. In the laboratory, nylon 6,6 can also be made using adipoyl chloride instead of adipic acid.
Biological macromolecules
The best known biological macromolecules are deoxyribonucleic acid (DNA), ribonucleic acid (RNA), proteins and polysaccharides. There is a huge diversity of these molecules in living organisms, and not all of their functions are completely known. All of them have a polarity and are synthesised by condensation reactions that add monomers to a defined end of the polymer. The (biological) chemistry and chemical functions of biological macromolecules are discussed further in Biochemistry.
Polypeptide
While nylon is a famous example of a synthetic polypeptide, in a biological context this class of macromolecules is generally referred to as "proteins". In proteins, the amide bond is known as a peptide bond and consists of the following pairing: left-chain -CO-NH- right chain (the C and the O are bound by a double bond, the N and H with a single bond, the combination is a carbonyl group). The amino acid residues that repeat to make large polypeptides are all linked by peptide bonds to form the primary sequence of the protein. The first amino acid in a polypeptide sequence is at the carboxy-terminal and the amino acid monomers are added to the amino-terminal using the messenger RNA molecules as a template to ensure the correct sequence. This transfer of information from the RNA molecule to synthesise the polypeptide is catalysed by the ribosome.
It must be pointed out that enzymes are a class of proteins with a catalytic function. Some other proteins have a more structural role, forming the ropes and tubes that are the framework of a cell (cytoskeleton) and the basis for locomotion in muscles. Plant proteins are also used for the storage of food in some seeds (maize and soyabean) and are a staple food group for most vegetarians.
Polynucleotide
This class of macromolecules includes the RNA and DNA molecules that store the information required to build a cell. The macromolecule has a repeating backbone of ribose sugars that are joined by phosphodiester (O-P-O) bonds. For DNA, each ribose has one of four bases (G, A, T and C) attached to complete the structure of the repeating nucleotides. The sequence of these four nucleotides is the chemical basis for the genetic code. Because the exact sequence must be conserved for the code to be maintained these macromolecules are synthesised by copying a template. This is called "transcription" for RNA and "replication" for DNA; both processes use the genomic DNA sequence as the template.
Polysaccharide
Polysaccharides are the simplest biological macromolecules, often consisting of only one repeating glucose unit joined by glycosidic bonds. In animals, these macromolecules are primarily for storage, but they have an important structural role in plants, as cellulose is the primary component of their cell walls. In the form of cotton fibers, cellulose is an important textile raw material.
Although cellulose is the most abundant macromolecule on Earth, it is of limited use to animals as a food source because of the lack of an appropriate enzyme to cleave the bond between glucose monomers ( β 1,4 glycosidic bond in cellulose) . Ruminants, such as cows, can digest cellulose in their second stomach by co-opting bacteria to digest the cellulose. Common polysaccharides that are digestible by animals are starch and glycogen and both employ a different kind of bond between the glucose monomers (termed an α 1,4 glycosidic bond). Animals do have enzymes that can hydrolyse the α 1,4 glycosidic bonds of these polysaccharides to release the glucose monomers.
Polyhydrocarbon
A lipid is synthesized by adding two fatty acid chains (polyhydrocarbons) to a three carbon backbone. In cells, the third carbon is often a polar head group, linked by a phosphate, such that these phospholipids have a hydrophobic fatty acid tail and a hydrophilic head. In an aqueous environment the most stable conformation for the phospholipids is to form a bilayer with the hydrocarbon tails interacting with each other and the polar head group interacting with the surrounding water. Phospholipid bilayers are the basis for cellular life, as the double layer of lipids is the key component of cell membranes that compartmentalize cellular metabolism in a controlled environment.
Cell membranes are also integrally associated with proteins, some of which pass directly through the membrane. The function of the whole membrane structure is diverse and includes signaling, transport and metabolism, all of which depend on the fluidity of the proteins and lipids in the membrane. Their movement (reaching up to speeds of 350 ms-1) over a broad range of temperatures is made possible by cholesterol, a substance needed to make the membrane more fluid-like at low temperatures and yet less fluid-like at high temperatures.
Introduction to Physical polymer chemistry
- The subtopics of Physical polymer chemistry are Statistical physics, Statistical thermodynamics, Graph theory, Group Theory, Monte Carlo simulations, Scaling theory, Physics of polymer solutions, Stochastic processes and Chemistry of polymer solutions.
The physical properties of a macromolecule depend largely upon its composition. A simple hydrocarbon chain has a 'skeleton' of carbon atoms to which hydrogen atoms are attached; the general composition of a pure hydrocarbon is CnH2n+2, where all bonds between the carbon atoms have been completely saturated (by hydrogen). The simplest hydrocarbon is methane (CH4); methane is a greenhouse gas, and is highly inflammable and explosive. The next largest hydrocarbons, ethane, propane and butane, are also gases at room temperature (20˚C), and propane and butane are widely used as fuels for heating. As can be deduced from their composition, hydrocarbons with longer chains than butane tend to be liquid at room temperature, and very high chain length hydrocarbons are solids.
Because of the simple composition of the first four hydrocarbons and their small molecular sizes, the lack of any significant internal polarity makes it hard for these molecules to interact either with one another or with the molecules of a solvent. In water, therefore, these molecules will escape from solution and diffuse into the air. Larger hydrocarbon molecules will shy away from the solvent water and will form a double layer system of water and oil. The thorough mixing of these two layers with the help of an emulsifier into a stable emulsion is a basic ingredient of cooking and many cosmetic products. Anyone who has tried to mix an oily ingredient with a watery ingredient will know of the separation of phases (for instance when trying to make mayonnaise), but easy home experiments (better known as cooking) can solve that problem of phase separation.
Any charged polyelectrolyte can be treated like a usual ionic electrolyte. When the macromolecule is acidic, the polymeric acid can be titrated using a litmus test to determine its "concentration". In this case, the "concentration" is now somewhat of a statistical answer, as the weight of the molecule and its molecular weight are only known within statistical limits. The concentration of macromolecules is also a statistical mean value for that reason. Like a simple solution, macromolecules dissolve in the right solvent and show the same interactions with the solvent. In an aqueous solution, the hydrophilic parts of a macromolecule will be - on average - more exposed to the aqueous solvent than its hydrophobic parts. Using these hydrophilic and hydrophobic qualities DNA gets transcribed by transcriptases binding to the DNA in electrodynamical ways, partly hydrogen bonding plays a role as well as local substrate concentration. A functioning DNA molecule reacts in a way that chemists can describe relatively simply, such as by its kinetics, reactivity and physico-chemical behavior. However, the biological implications may not always be easy to understand.
Macromolecules do exhibit different behaviour as well. Physical chemists use light diffraction (direct and indirect - or first- and second-order light diffraction) to determine the shape of a molecule, just as a rotating cigar will diffract light differently to a rotating ball. Shape can be a clue to the functionality of a molecule in vivo. Similar results can be obtained from viscosimetric observations, where the shape of a molecule in terms of its hydrodynamic radius can be determined by comparing the behavior of the solvent to that of the solution. These results can be calculated from equations given in some of the textbooks below.
Another way to describe the behavior of macromolecules is by using stochastics as the mathematical description of observed behavior. The conformation of a macromolecule in a solvent can be described as a stochastic process with the configuration as the leading part and the electric behavior (e.g. amount of charges, occurrences of hydrogen bonds, the nature of the interactions between the solute and the solvent) as a series of smaller, fast fluctuating terms as a function of time. This description leads the way to numerical analyses, and is one of the ways used in Monte Carlo simulations. The numerical answer can be used to help explain some of the hydrodynamic aspects and behavior of the macromolecule.
Unsaturated hydrocarbons
Reducing the hydrogen content of a completely hydrogenated hydrocarbon amounts to introduction of sp2 hybridization by removing two H atoms and forming a double valence bond between the two adjacent C-atoms. This "double bonded" C-C bond is more rigid than a single bond, and is in the same plane as the resulting C-H bonds. This means that the hydrocarbon becomes more rigid, and greater rigidity occurs when there are more of these double bonds in the molecule. This rigidity affects the physical properties of the molecule, such as its shape, solubility and interactions. When using unsaturated hydrocarbons, it is useful to know how the chain is composed and how the functional groups are connected to the molecule. For that reason, the cis- and trans- term was introduced. Cis-hydrocarbonates have the primary chain in a bent way. The functional group between two double-bonded carbon atoms sticks out in the same plane, whereas with trans-hydrocarbonates the functional groups are on either side of the chain. [3] The chemical reactivity of cis and trans hydrocarbonates can differ however, without the chemical formula being changed, as configuration is important here. This can be compared to naturally produced saccharides, their stereo-isomers always rotate light to the right, not to the left, whereas man-made saccharides always come in a racemic, or optical neutral, mixture of both left- and right-rotating saccharides.
Introducing functional groups
Introducing other functional groups onto the hydrocarbon chain brings new physical and chemical behavior. Replacing one H by a phenyl-group or toluene makes the molecule more extended in space and less flexible, again depending on how many of these replacements there are. If on the other hand the replacement group is more hydrophilic, completely new behavior can arise, such as increased solubility in water. Macromolecules with many acidic or basic group replacements are called polyelectrolytes; as with small electrolytes, dissolving them will produce charged ions. In the case of acids or bases, dissolving the polymer releases either protons H+ or OH−-ions. This concept of acid-base reactions can be applied directly to polyelectrolytes, as illustrated by the presence of acidic or basic functional groups in the molecule. Additionally, an 'anionic' functional group on the polymer must have an associated 'cation', and vice versa. It is like a solution of NaCl. There need to be equal amounts of the positively charged Na+ as the negatively charged Cl−, since the overall solution is not carrying any charges. A carboxylic functional group can have a metal ion associated with it, balancing the charges. Or poly (acrylic) acid, when charged (partially titrated) with sodium hydroxide NaOH, has the acidic groups (HCO2−) paired with a Na+ ion with it.
Scalability and Scaling theory
Scaling theory applied to macromolecules has led to the idea of repetitive units that each contribute to the overall behavior of a macromolecule. These units are not necessarily the same as the repeating monomeric unit when calculated from the longer chain polymer molecule. For instance, when the chemist uses the monomeric unit as the building block, statistical methods lead to a different length scale than for a repetitive unit. Or there is a different electrochemical scale where individual charges are scaled according to the observed properties of the macromolecule. A long chain of hydrocarbon with the general formula CnH2n+2 has considerable rotational freedom; all the bonds in the molecule can freely rotate, and even bend to some extent. In solution, for a simple molecule such as a pure hydrocarbon, the monomeric unit is most important in determining the properties of the molecule. When functional groups are introduced, they influence the rotation of bonds, purely by their 3-dimensional size; because of the size of these groups, the molecule can rotate less freely on its internal bonds, and so will seem to be more rigid. Overall, the effect is that the molecule (based upon its rigidity) has fewer repetitive units than monomeric units, when related to physical properties such as hydrodynamic radius, rheological behavior and even electrical behavior. This behavior contributes to the statistical physical properties of dilute and very dilute solutions. Another example is electrostatic shielding of charges on partially charged polyelectrolytes by hydrophobic, non-polar functional groups. Statistically, the charge per unit scales in a way similar to the effective length of a monomeric unit (persistence length). In calculating properties from fundamental molecular structure, the electron charge e is more accurately replaced by the effective electric charge e*. The effective charge can have a considerable influence on the conformational properties of macromolecules, i.e. molecules act as if they contain more or less charge on their backbone (depending on the particular circumstances), with subtle effects of electrostatic repulsion on molecule behavior.
Persistence length
One of the most obvious characteristics of macromolecules is their rigidity (stiffness). This is measured by the persistence length, which can be obtained by physical statistical analysis. The longer the persistence length, the more rigid a macromolecule is in its behavior and characteristics. The persistence length can be seen as the average of the length, scaled to the property of interest to be measured or determined. It allows a better understanding of behavior of molecules in different circumstances.
To visualize the persistence of a macromolecule, we might picture it as an assembly of rigid bars joined by connections that allow them to rotate freely in all three dimensions. This is the model one can apply to a long hydrocarbon with the chemical formula CnH2n+2. Introducing more spacious functional groups into the molecule affects the freedom with which the bars can rotate. The overall result is that, for purposes of calculation, the length of these bars must be replaced by another length to obtain the same formulas and results. This "new" length is the persistence length. The more limited the free rotation is, the larger that length will become - reflecting the increased rigidity of the molecule.
Functions of macromolecules
Engineering macromolecules to display a specific behavior is now a common process in the pharmaceutical industry. In biotechnology, similar processes are used to create molecules with pre-defined properties, often for their pharmaceutical benefits. Computational chemists and biochemists are trying to reconstruct molecules that mimic the behavior of natural molecules,in order to study how and why their behavior follows from the composition and the conformation. So-called "intelligent polymers" change their properties (for example their shape, surface characteristics or solubility) in response to small changes in their environment. For example, poly(N-isopropylacrylamide) (poly(NIPA)) is temperature-sensitive; the polymer chains change from water-soluble coils to water-insoluble globules in aqueous solution when the temperature rises above a threshold level. Intelligent polymers have been used, amongst other things, for drug delivery, tissue engineering, and bioseparation.[4]
The best known example of how the function of a macromolecule relates to its composition is hemoglobin. Hemoglobin is a metalloprotein in the red blood cells of vertebrates that transports oxygen from the lungs or gills to the tissues of the body. Hemoglobin has four separate chains of protein (four subunits). Due to specific hydrogen bonding, each chain forms a molecule with a cavity that can specifically bind Fe3+ ions. It is these four iron ions that bind oxygen tightly in the oxygenated blood of the lungs, yet, release the cargo in blood with lower oxygen levels where it is consumed by the surrounding cells.
The composition of cellular components is often a mix of several different macromolecules working cooperatively. Ribosomes, the 'cellular machines' required for synthesizing polypeptides, are formed from tens of different proteins interacting with at least three different polynucleotides. The phospholipid bilayer acts together with saccharides, proteins and cholesterol to make the functional membrane that encloses every biological cell—the basic unit of all living things.
The configuration of a macromolecule is the default configuration of the class of macromolecules that it belongs to. It excludes functional sites and functional groups in the case of enzymes, or behavior anomalous for that class of macromolecules. It describes the generic 3-D configuration of a molecule without its interaction with the actual surroundings.
By contrast, the conformation of a macromolecule is its (chemical) behavior in the environment in which it is observed. Often, that behavior is determined by the configuration of the molecule as changed by specific components, either in the molecule or in its environment, that affect its structure, behavior and responses. For example, the configuration of a DNA molecule is of a double-stranded α-helix. By contrast, its conformation, when not in a particular active mode, is that of an imploded, compact, super-coiled molecule, nearly oblivious to its environment, until it receives an awakening 'call'.
This same difference between configuration and conformation can be seen in many macromolecules. Some behave as if they 'remember' their old shape, returning to it when put under 'a stress'. Partially charged polyelectrolytes form a loosely-wound knot (like a very loosely-wound watery knot of wool), fluctuating in density and configuration during its normal state as being the solute. Many polyelectrolytes bond very strongly to metal ions, generally producing tightly bound coils with the metal ion in its middle and hydrophilic groups exposed to the exterior, most likely a watery solution. When combined with another solution containing metals, they tend to form 'cages'—sometimes very tightly bound cages—around these metal-ions, that precipitate during the reaction. Similar properties for poly(acrylic) acid (PAA), that can selectively bind heavy metals, are exploited for environmental cleanup. After treating silt the precipitate is rich in heavy (poisonous) metals, such as Cd, Cu and other toxic metals such as Pb and Hg, either in their metallic form or as tightly bound salt.
Biological catalysts or enzymes show the same behavior as other catalysts, except that most biological catalysts need a particular configuration or folding in three dimensions to be effective. Even slight aberrations from this configuration can dramatically affect their activity. Many proteins even exploit these conformation changes to switch between 'on' and 'off' states. For example, in the brain, signals are carried by a wide variety of molecules that interact with polypeptide receptors on the surface of neuronal cells. The receptors are macromolecules that span the cell membrane; on the outside of the cell, the receptor forms a 'pocket' that binds specific signaling molecules and this initiates a change in the receptors conformation. This change can propagate across the membrane and affect the function of the receptor molecule inside the cell, often starting a signaling cascade. Compare it to activating an alarm system using a central key, it affects all areas controlled by that alarm system—signaling those parts of importance with the needed and appropriate information.
In some cases, how a molecule behaves cannot be clearly attributed to either conformation or functional groups alone, as these two features seem to 'cooperate' in their contributions. Examples include many enzymes and biological systems involved in for instance photosynthesis, transport of nutrients into cells, the responses of cells to stimuli, and synaptic connections between neurons in the nervous system. Neurons make many different receptor molecules that are present in the cell membrane. These molecules bind specific messenger substances, and when these messengers bind to the receptor molecule, this triggers a biochemical response within the neuron. The binding of a messenger substance to a receptor molecule depends on both the physical shape of the receptor molecule and its composition.
References
- ↑ The Petpower website
- ↑ Sources of raw material
- Genencor International and DuPont Expand R&D Collaboration to Make Key Biobased Polymer
- DuPont Engineering Polymers Previews Plans to Produce a New Family of High-Performance Polymers Made with Renewable Resources
- Polylactide polymers at NatureWorks website Accessed 3 February 2007
- Vink ETH et al. (2004) [1] The sustainability of NatureWorksTM polylactide polymers and IngeoTM polylactide fibers: an update of the future. Macromolecular Bioscience 4:551-64 DOI:10.1002/mabi.200400023
- ↑ Note that this has no connection with the trans-fat name used now in a variety of health-related items.
- ↑ Piskin E (2004) Molecularly designed water soluble, intelligent, nanosize polymeric carriers. Int J Pharm 277:105-18 PMID 15158974